- 1Instituto de Biología Celular y Neurociencia “Prof. E. De Robertis” (IBCN), Universidad de Buenos Aires-CONICET, Buenos Aires, Argentina
- 2Biomarkers and Molecular Signaling Group, Center for Biomedical Research of La Rioja (CIBIR), Logroño, Spain
- 3Angiogenesis Study Group, Center for Biomedical Research of La Rioja (CIBIR), Logroño, Spain
In the last few years, an increasing interest in the neuroprotective effect of cannabinoids has taken place. The aim of the present work was to study the effects of modulating cannabinoid receptor 1 (CB1) in the context of light induced retinal degeneration (LIRD), using an animal model that resembles many characteristics of human age-related macular degeneration (AMD) and other degenerative diseases of the outer retina. Sprague Dawley rats (n = 28) were intravitreally injected in the right eye with either a CB1 agonist (ACEA), or an antagonist (AM251). Contralateral eyes were injected with respective vehicles as controls. Then, rats were subjected to continuous illumination (12,000 lux) for 24 h. Retinas from 28 animals were processed by GFAP-immunohistochemistry (IHC), TUNEL technique, Western blotting (WB), or qRT-PCR. ACEA-treated retinas showed a significantly lower number of apoptotic nuclei in the outer nuclear layer (ONL), lower levels of activated Caspase-3 by WB, and lower levels of glial reactivity by both GFAP-IHC and WB. qRT-PCR revealed that ACEA significantly decreased the expression of Bcl-2 and CYP1A1. Conversely, AM251-treated retinas showed a higher number of apoptotic nuclei in the ONL, higher levels of activated Caspase-3 by WB, and higher levels of glial reactivity as determined by GFAP-IHC and WB. AM251 increased the expression of Bcl-2, Bad, Bax, Aryl hydrocarbon Receptor (AhR), GFAP, and TNFα. In summary, the stimulation of the CB1 receptor, previous to the start of the pathogenic process, improved the survival of photoreceptors exposed to LIRD. The modulation of CB1 activity may be used as a neuroprotective strategy in retinal degeneration and deserves further studies.
Introduction
Endocannabinoids (eCBs) are responsible for a wide range of physiological reactions that include modulation of synaptic function, control of tumor growth, analgesia, appetite stimulation, reduction of nausea, psychotropic effects, and synaptic plasticity, among others (1–4). The two most studied eCBs are N-arachidonoylethanolamide or anandamide (AEA) and 2-arachidonoylglycerol (2-AG). AEA is synthesized by phospholipase D (NAPE-PLD) from N-arachidonoyl phosphatidylethanolamine (NAPE) while 2-AG is synthesized by two diacylglycerol-lipase isoenzymes, DAGLα and DAGLβ. After binding to their receptors, eCBs are inactivated mainly by the fatty acid amide hydrolase enzyme (FAAH) and, to a lesser extent, by monoacylglycerol lipase (MGL), cyclooxygenase-2 (COX-2), and lipoxygenase (LOX) (5).
The effects of eCBs are mediated by metabotropic receptors (CB1 and CB2) and ionotropic receptor TRPV1. CB1 is the most abundant G-protein coupled receptor (GPCR) in the CNS. CB2 is also a GPCR that has been described in peripheral tissues, mainly in the immune system, but it has also been reported in the CNS including the retina (6–8). The activation of these receptors decreases the release of GABA and glutamate. TRPV1 is widely distributed in the CNS and, to a lesser extent, in the periphery. In addition, eCBs are ligands of peroxisome proliferator-activated receptor (PPAR-γ), which is involved in lipid metabolism, insulin sensitivity, regulation of inflammation and proliferation (9).
CB1 receptor and FAAH have been reported in the retina of rodents and primates (10, 11). High levels of CB1 receptor have been shown in the retina, iris and ciliary body of the human eye (12). The CB1 receptor and the degradative enzymes are mainly concentrated in the projection glutamatergic pathway. The CB2 receptor was reported in the retinal pigment epithelium (RPE), in photoreceptors, in the inner nuclear layer and in ganglion cells of the retina (13, 8). In the retina, cannabinoids regulate neurotransmitter release and modulate the retinal response to light. These results were supported by alterations of electroretinogram recordings in mice lacking CB1 and CB2 cannabinoid receptors (14).
Cannabinoid receptors protect CNS neurons from oxidative damage (15). In this regard, changes have been reported in eCB levels in diabetic retinopathy and age-related macular degeneration (AMD) (16). Cannabidiol significantly reduced both oxidative stress and neurotoxicity, and prevented retinal cell death in a rat model of diabetic retinopathy (17). Mixed agonists, which activate both CB1 and CB2, have a neuroprotective role in NMDA-induced excitotoxicity (18). Other studies have verified the protective role of the CB1 and TRPV receptors in glaucoma and retinal ischemia (19, 20). eCB agonists have being shown to exert a protective role in another model of glaucoma (21). The neuroprotective role of cannabinoids was also shown in an animal model of autosomal dominant retinitis pigmentosa (22).
Light induced retinal degeneration (LIRD) has been widely used as an animal model to study degenerative diseases of the retina (23–28). Continuous illumination (CI) produces photoreceptor degeneration, apoptosis in the outer nuclear layer (ONL), and synaptic degeneration in the outer plexiform layer (24, 29, 30, 31, 32, 33). The degenerative process starts in the outer retina, as happens in human AMD, juvenile macular degeneration, and retinitis pigmentosa (34). AMD is the first cause of acquired blindness in developed countries (35) and the majority of patients require indefinite treatment with antiangiogenic drugs or demonstrate disease progression despite therapies (36). This animal model is useful to study the potential neuroprotective effect of new drugs (37, 38). The aim of the present work was to study the effects of modulating the CB1 receptor in the LIRD model, in order to explore new therapies for AMD and other degenerative diseases of the outer retina.
Materials and Methods
Animals
Male Sprague Dawley albino rats (n = 28, body weight 200 g, age 60 days) were used. Animal care was performed in accordance with the Association for Research in Vision and Ophthalmology Statement for the Use of Animals in Ophthalmic and Vision Research. The animal model of continuous illumination and the experimental procedure was approved by the Institutional Committee for the Use and Care of Laboratory Animals of the Facultad de Medicina, UBA [CICUAL, Res. (CD) 3130/2017].
Intravitreal Injections Protocol
Intravitreal injections were performed as previously described (37, 38). Animals were anestethized with Ketamine (40 mg/kg; Ketamina 50®, Holliday-Scott SA, Argentina) and Xylazine (5 mg/kg; Kensol®, König SA, Argentina). A drop of 2% lidocaine (Lidocaine®, Richmond SA, Argentina) was administered to each eye. Intravitreal injections (5 µl) were performed using a Hamilton syringe (Reno, NV, United States) and a 30-gauge needle. The right eyes received either ACEA (Sigma-Aldrich, Cat #A9719), a CB1 agonist, or AM251 (Sigma, Cat #A6226), a CB1 antagonist. The left eyes were used as controls (CTL) and received the same volume of vehicle (0.001% DMSO in 0.9 g/l NaCl). The final vitreal concentrations were 10 μM for ACEA and 2 μM for AM251. Doses were selected based on a previous scientific study (39).
Continuous Illumination Procedure
After intravitreal injections and once animals recovered completely from anesthesia, rats were continuously illuminated for 24 h at 12,000 lux as previously described (37, 38). Illumination procedure was initiated during daylight period at 2 p.m. approximately. Groups of 3–5 rats were simultaneously placed in an open white acrylic box of 60 cm × 60 cm x 60 cm with 12 halogen lamps (12 V, 50 W each) located on top. Lighting level (12,000 lux) was determined using a digital illuminance meter. Temperature was maintained at 21°C and animals were offered food and water ad libitum. Immediately after completing the illumination protocol, 28 rats were sacrificed and their retinas were processed for qRT-PCR (n = 10), GFAP immunohistochemistry (IHC) and TUNEL technique (n = 8), or Western blotting (WB) (n = 10).
Tissue Processing for Immunohistochemistry and TUNEL Assay
Rats were deeply anaesthetized by intraperitoneal injection of Ketamine and Xylazine as mentioned before and their eyes were removed. The cornea and lenses were cut off, and the remaining tissues were fixed by immersion in a solution containing 4% paraformaldehyde for 24 h. Eyes were embedded in paraffin and sectioned along a meridional plane in a Leica RM2125 RTA microtome (thickness: 5 µm). Prior to IHC or TUNEL, sections were subjected to an antigen retrieval protocol:Tris-EDTA Buffer (pH 9.0) at 90°C for 30 min.
Immunoperoxidase Technique
Sections were incubated in methanol containing 3% hydrogen peroxide for 30 min in order to inhibit endogenous peroxidase activity. After washing in phosphate buffered saline (PBS), pH 7.4, sections were incubated in 10% normal goat serum for 1 h. Then, sections were incubated overnight with GFAP polyclonal primary antibody (Dako, Cat #Z0334, United States, dilution 1:500) at 4°C. The following day, sections were incubated sequentially in biotinylated goat anti-rabbit antibody (Sigma Chemical Co., MO; Cat #B8895, dilution 1:500) and in ExtrAvidin-Peroxidase® complex (Sigma Chemical Co., MO., Cat #E2886, United States; dilution 1:500) at room temperature (RT) for 1 h. Development was performed using the DAB/nickel intensification procedure (40). Controls were performed by omitting primary antibodies (Supplementary Figure S1). Also retinas from non-illuminated and illuminated rats for 24 hs were stained by GFAP IHC as additional controls (Supplementary Figure S1).
Terminal Deoxynucleotidyl Transferase dUTP Nick End Labeling Assay
Sections were processed using the ApopTag® Peroxidase In Situ kit (Millipore, United States). Briefly, sections were washed in PBS and post-fixed in ethanol:acetic acid (2:1) at −20°C. After washing in PBS the endogenous peroxidase was inhibited as mentioned above. Then, sections were incubated sequentially with terminal deoxynucleotidyl transferase (1 h at 37°C) and with anti-digoxigenin conjugate (30 min at RT). Development was performed using the DAB/nickel intensification procedure followed by eosine counterstaining.
Image Analysis of TUNEL and GFAP Immunoperoxidase Sections
Six retinal sections of both eyes from each experimental group were analyzed (ACEA, n = 4; AM251, n = 4). Anatomically matched areas of retina among animals were selected and. images were taken using a Zeiss Axiophot microscope attached to a video camera (Olympus Q5) under the same light conditions.
The following parameters were measured, blind to treatment, on 8 bits images, using the Fiji software (NIH, Research Services Branch, NIMH, Bethesda, MD):
GFAP positive area: Images of drug treated and control retinas were randomly selected. Immunoreactive area of the whole sections was thresholded. The region of interest (ROI) was the retinal surface between the two limiting membranes where Müller cells extend their processes. The GFAP positive area was calculated as the percentage of the ROI immunostained by GFAP.
TUNEL positive nuclei/1000 µm2: Images of drug treated and control retinas were randomly selected and thresholded. As ROI, frames of 1000 μm2 were randomly determined on the ONL of treated and control retinas. The “analyse particles” function of Fiji was used and the TUNEL positive nuclei/1000 µm2 ratio was then obtained for each ROI.
Western-Blotting
Retinas were homogenized (1:3, w/v) in lysis buffer (100 mM NaCl, 10 mM TrisHCL, 0.5% Triton X-100) plus 50 µl of Protease inhibitor cocktail (Merck KGaA, Darmstadt, Germany) at 4°C. Protein concentration was determined by the Bradford method. Then, 50–100 µl of each sample were mixed 4:1 with 5X sample buffer (10% SDS, 0315 M Tris-HCl, 25% beta-mercaptoethanol, 50% glycerol, 0.2 ml bromophenol blue 0.1%, pH 6.8), separated by 15% SDS–PAGE and transferred to polyvinylidenedifluoride membranes (GE healthcare life sciences, IL). Kaleidoscope Prestained Standards (Bio-Rad Laboratories, CA) were used as molecular weight markers. Membranes were blocked with PBS/5% non-fat dry milk and incubated overnight at 4°C with either a rabbit polyclonal antibody to GFAP (DAKO Inc., CA, United States; Cat #Z0334, dilution 1:500) or a rabbit polyclonal antibody to activated Caspase-3 (Sigma Chemical Co., MO, United States; Cat #H277, dilution 1:100) or a monoclonal anti-β-actin antibody (Sigma Chemical Co., MO, United States, Cat #C8487, dilution: 1: 1000). Membranes were incubated with ECL donkey anti-rabbit IgG, HRP-linked F (ab)2 fragment (Amersham), and were developed using a chemoluminiscence kit (SuperSignal West Pico Chemiluminescent Substrate, Thermo Scientific, MA). Membranes were exposed to X-ray blue films (Agfa Healthcare, Argentina), which were developed and then scanned with a HP Photosmart scanner (Hewlett Packard). Optical density was quantified by the Image Studio Light software of Li-Cor.
RNA Isolation and Quantitative Reverse Transcription Polymerase Chain Reaction
The retinas of CTL and drug-treated retinas (ACEA and AM251, n = 5 per group) which were subjected to 24 h of continuous illumination were dissected out. Procedure was performed as detailed in Soliño et al. (37). Briefly, retinas were homogenized with TRIzol (Invitrogen, Madrid, Spain) and RNA was isolated with RNeasy Mini kit (Qiagen, Germantown, MD). Three µg of total RNA were treated with 0.5 µl DNAseI (Invitrogen) and reverse-transcribed into first-strand cDNA using random primers and the SuperScript III kit (Invitrogen).
Reverse transcriptase was omitted in control reactions. Resulting cDNA was mixed with SYBR Green PCR master mix (Invitrogen) for qRT-PCR using 0.3 µM forward and reverse oligonucleotide primers (Table 1). Quantitative measures were performed using a 7,300 Real Time PCR System (Applied Biosystems, Carlsbad, CA). Cycling conditions were an initial denaturation at 95°C for 10 min, followed by 40 cycles of 95°C for 15 s and 60°C for 1 min. At the end, a dissociation curve was implemented from 60 to 95°C to validate amplicon specificity. Gene expression was calculated using relative quantification by interpolation into a standard curve. All values were divided by the expression of the house keeping gene 18S.
Statistical Analysis
The image data analysis of GFAP IHC and TUNEL studies of AEA-treated rats (n = 4) and AM251-treated rats (n = 4) were evaluated using D´Agostino, KS, Shapiro-Wilk, and F tests. In every case, Gaussian distribution was confirmed. Then, data were analysed using unpaired Student´s t-test (GraphPad Software, San Diego, CA). The results of WB (n = 5, per drug treatment), and qRT-PCR (n = 5, per drug treatment) were analysed using unpaired Student´s t-test. In every case, values are expressed as mean ± standard deviation. Differences were considered significant when p < 0.05.
The sample size was calculated based on data published by Soliño et al. (37). Free software (http://biomath.info/power/ttest.htm) was used to calculate the sample size. Power was set as 80% for an alpha of 5%, resulting in less than six animals per group to reach a significant improvement of the variable with an unpaired t-test.
Results
Effects of the Administration of ACEA on Light Induced Retinal Degeneration
Effects on Photoreceptor Apoptosis and Gliosis by Morphological Techniques
The quantification of apoptotic cells by TUNEL technique showed that treatment with ACEA decreased cell death. The eyes treated with ACEA had lower numbers of TUNEL positive nuclei in the ONL than the CTL eyes (11.01 ± 1.62 vs. 16.31 ± 1.98, p = 0.0041, n = 4) (Figure 1).
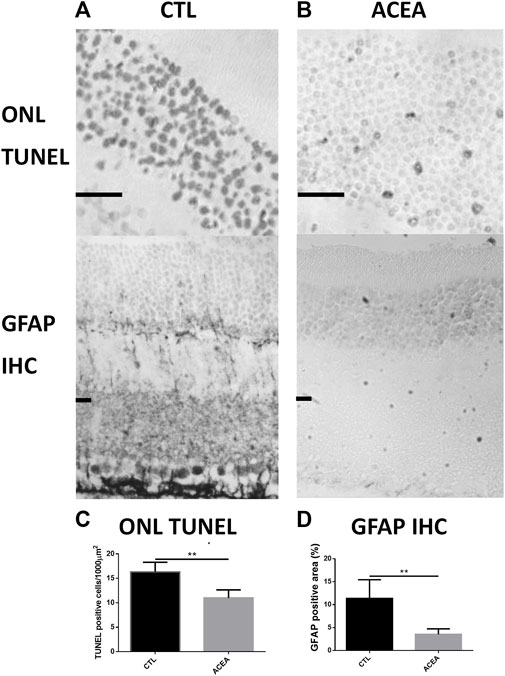
FIGURE 1. Treatment with ACEA decreased the number of apoptotic nuclei and GFAP-immunoreactive areas. (A): Representative TUNEL stained sections of the outer nuclear layer (ONL) of the retina of a CTL eye (Left) and an ACEA-treated eye (Right). Observe the higher amount of apoptotic nuclei in the CTL eye compared to the ACEA-treated eye. Scale bar: 20 μm. (B): Representative GFAP immunostained retinal sections of a CTL eye (Left) and an ACEA-treated eye (Right). A greater immunoreactivity of Müller cells is observed in the retina of the CTL eye compared to ACEA eye. (C): Quantification of ONL TUNEL positive cells. (D) Quantification of positive GFAP immunostained area. **p < 0.01.
Quantification of the GFAP immunoreactive area in the ACEA-treated retinas showed a significant decrease of the glial reactivity compared to CTL (3.53% ± 1.19 vs 11.37% ± 4.06, p = 0.0181, n = 4) (Figure 1 and Supplementary Figure S1).
Effects on Apoptosis and Glial Reactivity by Western Blot
WB showed significant differences between the ACEA-treated eyes and their controls in the levels of activated Caspase-3 (aC3) and GFAP. ACEA decreased significantly the expression of aC3 (0.87 ± 0.06 vs 1.00 ± 0.09, p = 0.0329) and GFAP (0.85 ± 0.11 vs. 1.00 ± 0.09, p = 0.0484) (Figure 2).
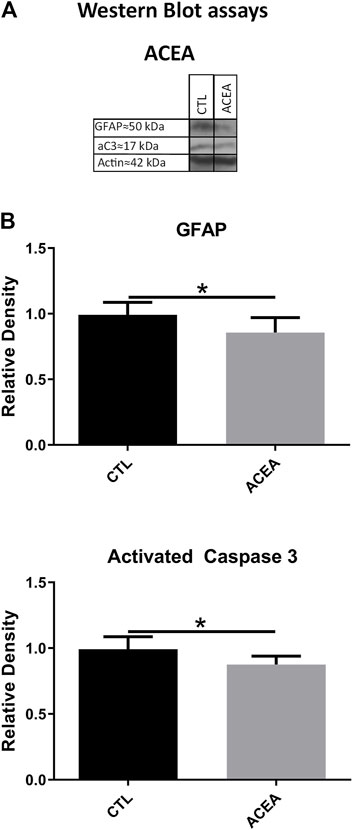
FIGURE 2. The treatment with ACEA decreased the levels of GFAP and activated Caspase-3 (aC3), (A): WB of retinas from CTL and ACEA-treated eyes. (B) quantifications of GFAP and aC3 bands, Means and standard deviations are shown. *p < 0.05.
Effects on Gene Expression (Quantitative Reverse Transcription-Polymerase Chain Reaction)
In order to investigate the mechanisms involved in retinal protection, the expression of genes involved in apoptosis, inflammation, and cell response to xenobiotics were studied. We studied the effects of the modulation of CB1 on the transcription of the pro-apoptotic proteins Bad and Bax and the antiapoptotic Bcl-2. ACEA treatment decreased Bcl-2 expression (p = 0.0283) (Figure 3), but did not significantly change the expression of inflammatory markers IL-1β, TNFα, and iNOS. GFAP expression was not modified either (Figure 4).
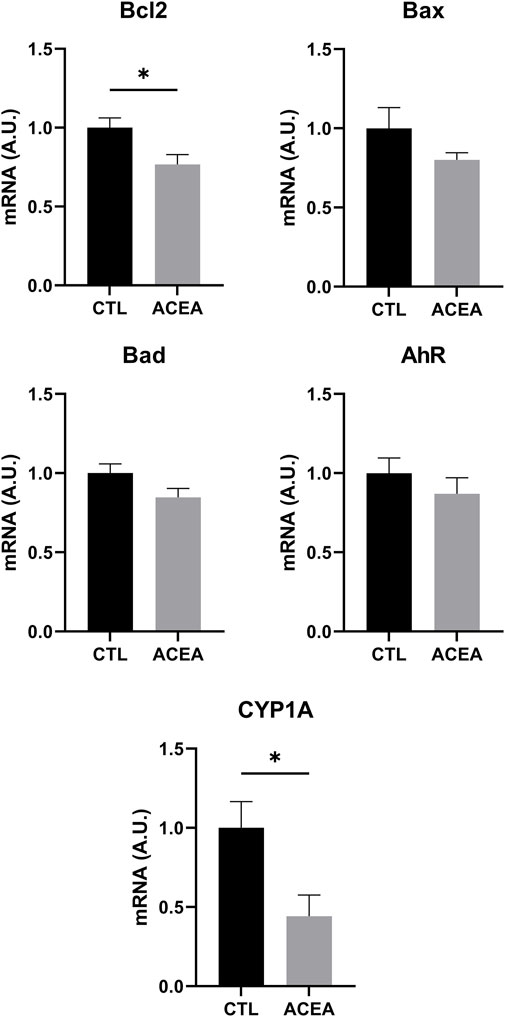
FIGURE 3. qRT-PCR of Bcl-2, Bax, Bad, AhR, and CYP1A1 of retinas from ACEA-treated eyes and their respective CTL. Means and standard deviations are shown. *p < 0.05.
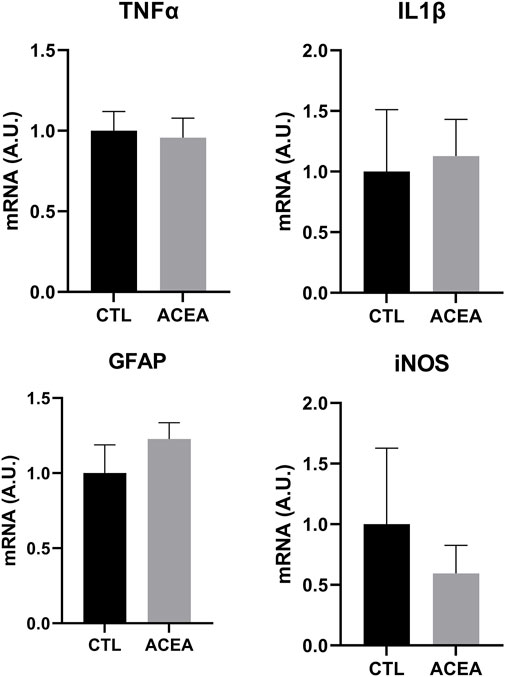
FIGURE 4. qRT-PCR of TNFα, IL1β, GFAP and iNOS from retinas of ACEA-treated eyes and their respective CTL. Means and standard deviations are shown.
The analysis of Aryl hydrocarbon Receptor (AhR), a transcription factor involved in the response to xenobiotics, and one of its target genes, CYP1A1, revealed that only CYP1A1was significantly decreased by ACEA treatment (p = 0.0307) (Figure 3).
Effects of the Administration of AM251 on Light-Induced Retinal Degeneration
Effects on Photoreceptor Apoptosis and Gliosis by Morphological Techniques
The quantification of apoptotic cells by TUNEL in AM251-treated eyes showed a significant increase in cell death compared with CTL eyes. The eyes treated with AM251 presented higher densities of TUNEL positive nuclei in the ONL (15.61 ± 3.13 vs 12.36 ± 2.30, p = 0.0096) (Figure 5). Also an increase of the percentage of GFAP immunoreactive area was found in the eyes that received AM251 compared to CTL eyes (15.16% ± 9.67 vs 8.08% ± 4.10, p = 0.0207) (Figure 5 and Supplementary Figure S1).
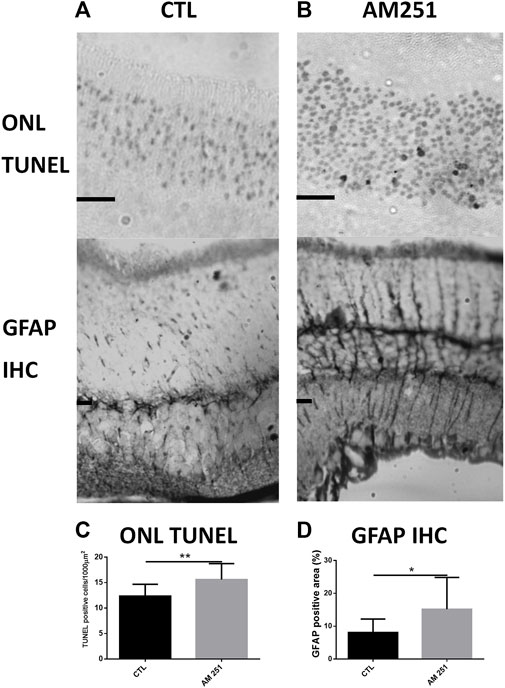
FIGURE 5. Treatment with AM251 increased the number of apoptotic nuclei and GFAP-immunoreactive areas. (A): Representative TUNEL stained sections of the outer nuclear layer (ONL) of the retina of a CTL eye (Left) and an AM251-treated eye (Right). Observe the higher amount of apoptotic nuclei in the AM251-treated eye compared to the CTL eye. Scale bar: 20 μm. (B): GFAP immunostained retinal sections of a CTL eye (Left) and an AM251-treated eye. A greater immunoreactivity of Müller cells is observed in the retina of the AM251-treated eye compared to the CTL eye. (C): Quantification of ONL TUNEL positive cells. (D) Quantification of positive GFAP immunostained area. *p < 0.05; **p < 0.01.
Effects on Apoptosis and Glial Reactivity by Western Blot
Intravitreal administration of AM 251 produced significant increases in the levels of GFAP (1.30 ± 0.18 vs. 1.00 ± 0.1, p = 0.0374) and aC3 (1.45 ± 0.47 vs 1.00 ± 0.10, p = 0.0441) when compared to the CTL eyes (Figure 6).
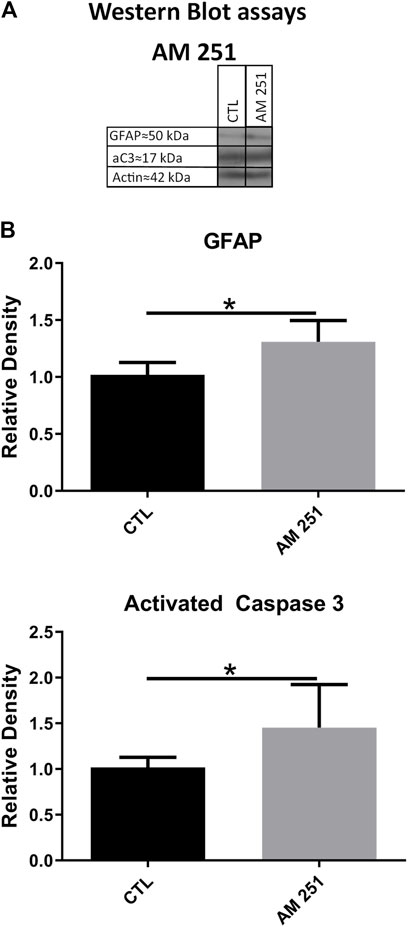
FIGURE 6. The treatment with AM251 increased the levels of GFAP and activated Caspase-3 (aC3). (A): WB of retinas from CTL and AM251-treated eyes. (B): Quantifications of GFAP and aC3 bands, Means and standard deviations are shown, *p < 0.05.
Effects on Gene Expression (Quantitative Reverse Transcription-Polymerase Chain Reaction)
AM 251 increased the mRNA of the apoptotic proteins Bad and Bax (Bax, p = 0.002 and Bad, p = 0.0095), but it also increased the mRNA for Bcl-2 (p = 0.0096) (Figure 7).
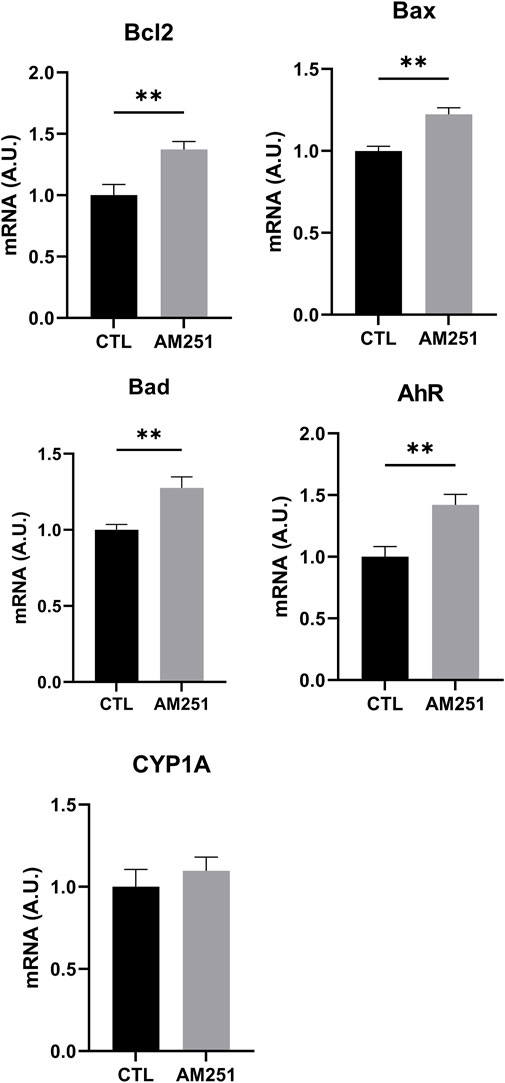
FIGURE 7. qRT-PCR of Bcl-2, Bax, Bad, AhR, and CYP1A1 of retinas from AM251-treated eyes and their respective CTL. Means and standard deviations are shown. **p < 0.01.
When we studied the expression of inflammatory markers, AM251 increased significantly TNFα (p = 0.0002) but it did not change IL-1β and iNOS levels. AM 251 also increased significantly the mRNA levels of GFAP (p = 0.0014) (Figure 8).
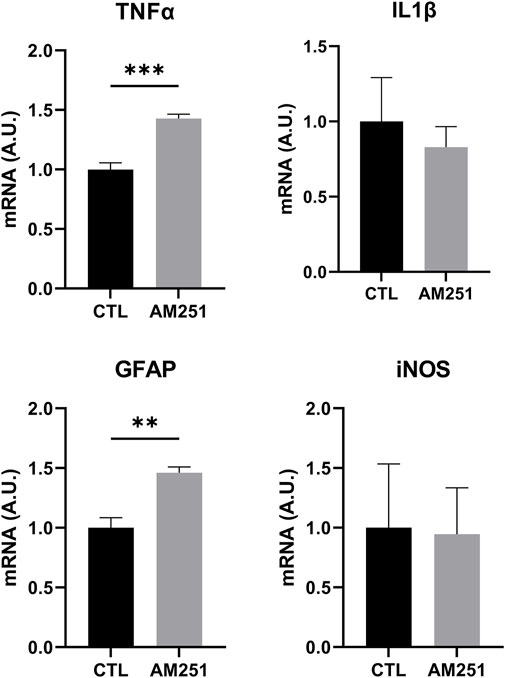
FIGURE 8. qRT-PCR of TNFα, IL1β, GFAP and iNOS from retinas of AM251-treated eyes and their respective CTL. Means and standard deviations are shown. **p < 0.01; ***p < 0.001.
In addition, AM251 increased significantly mRNA levels of AhR (p = 0.0074) (Figure 7).
Discussion
In our model, the intravitreal injection of ACEA, a CB1 receptor agonist, prior to LIRD proved to be neuroprotective. Although intravitreal injections may not be practical for the treatment of human AMD sufferers, it is a valued experimental approach that enabled us to achieve a known concentration of the drug in the tissue avoiding problems of absorption and pharmacokinetics. However, this is a route of administration of VEGF antibodies for the treatment of wet AMD.
We observed a lower number of photoreceptor apoptotic nuclei in the ONL and we detected lower levels of activated Caspase 3 by WB. In addition, lower levels of glial reactivity were determined by GFAP IHC and WB. Conversely, the intravitreal injection of AM251, a CB1 antagonist, prior to CI proved to be deleterious. In this case, we found a higher number of apoptotic nuclei in the ONL and higher levels of activated Caspase 3 by WB. This was accompanied by higher levels of glial reactivity determined by GFAP IHC and WB.
The qRT-PCR results indicated that CB1 blockade by AM251 caused an increase in the mRNA of IL1β, GFAP and of the proapoptotic proteins Bax and Bad. Surprisingly, the mRNA of the antiapoptotic factor Bcl-2 increased with AM251 and decreased with ACEA. An alternative explanation to these results could be that protein levels do not follow mRNA levels of Bcl-2. In the proapoptotic condition, under AM251 treatment, free Bcl-2 levels decrease as it heterodimerizes with Bad and Bax and so the synthesis of more Bcl-2 mRNA is stimulated and the opposite could happen in the anti-apoptotic condition under ACEA treatment. It is known that continuous illumination induces free radical production (27) and probably the treatment with AM251 produced a more harmful environment as shown by an increased expression of AhR. This receptor is activated by xenobiotics but it also participates in development, differentiation, proliferation, immune response and apoptosis (41). Its activation triggers the expression of cytochrome P450 enzyme CYP1A1 and also the expression of suppressor cytokine signaling-2 (SOCS-2) which modulates inflammatory response (42, 43). Supporting this idea, we found that ACEA-treated retinas showed a lower transcription of CYP1A1, a gene regulated by AhR. Probably the neuroprotective effect of CB1 stimulation arises from the final balance of a set of signals (combinatory regulation).
Our results are in agreement with other reports that show the neuroprotective effect of the eCB system in CNS using different models of Alzheimer disease, excitotoxicity, ischemia, and oxidative stress. The neuroprotective effect of CB1 signaling was demonstrated by different approaches. For instance, the protective effect of WIN55212–2, a CB1/CB2 receptor agonist, was blocked by a CB1 receptor antagonist (44) and CB1 receptor-deficient mice showed larger infarct areas and lower blood flow in the ischemic penumbra (45). The protective mechanism of CB1 receptor may involve survival pathways such as inositol triphosphate (IP3) (46), PI3K (45), ERK (47), the reduction of the activation on the nuclear factor-κB (48), and the inhibition of Q-type Ca2+ currents or the activation of inward K+ currents (49).
The cumulative effect of light produces free radicals (50). Then, reactive oxygen species (ROS) peroxide membrane phospholipids, and polyunsaturated fatty acids (PUFA) that belong to outer PR segments, modify relevant proteins involved in signal transduction and induce DNA damage triggering apoptosis (51–53). Oxidative stress may also induce mitochondrial dysfunction that leads to neurotoxicity. This inability to adapt to the environmental stressor (light) could result in metabolic inflexibility that leads to neurodegeneration (54). In fact, severe ultrastructural alterations were detected in mitochondria in our model (24). Also, mitochondria are probably involved in triggering apoptosis by releasing cytochrome c induced by the increase of Bad and Bax whose expression was enhanced after the treatment with AM251.
In the retina, using an NMDA-induced excitotoxicity model, it was shown that the treatment with phytocannabinoids decreased the stress produced by reactive nitrogen species and lipid peroxidation, acting partially through CB1 (18). In a model of axotomy of the optic nerve, it was found that inhibition of FAAH improved the survival of ganglion cells by a CB1-dependent mechanism that decreased microglial reactivity (55). Another possible site of action of CB1 in the retina could be the microvasculature. In this regard, it was found that the diameter of the capillaries and the size of the pericytes of the blood-retinal barrier are regulated by CB1 in concert with nitric oxide (NO) and the activation of guanylate cyclase (56).
Opposite to our results, using a model of retinal degeneration induced by intraperitoneal administration of N-methyl-N-nitrosourea (NMU), the treatment with SR141716A (Rimonabant), a CB1 antagonist, induced the survival of photoreceptors, lowered the levels of glial reactivity and decreased vascular anomalies (57). Similar results were also obtained in a diabetic mouse model of retinal degeneration where the deletion of CB1 receptor or the use of SR141716 (Rimonabant) prevented retinal cell death (58). Finally, using a model of continuous illumination, saffron and selective CB1 and CB2 antagonists also reduced photoreceptor death in ONL and preserved visual function of the retina (59). These differences with our results may arise from alternative neuroprotective properties of SR141716A, not necessarily related with CB1 antagonism. It has been suggested that this compound could have inverse agonist properties and may function as agonist on other receptors (60). An alternative explanation is that at the dose of 10 μΜ employed in our study, ACEA could activate the CB2 receptor. If fact, CB2 receptor agonists have been shown to be neuroprotective in animal models of EAE and stroke (61). In our laboratory, the use of JWH133, a CB2 agonist, protected photoreceptors from cell death in the same LIRD model (unpublished data).
In conclusion, in our hands, the stimulation of CB1 improved the survival of photoreceptors and decreased glial reactivity in the LIRD model. The modulation of CB1 activity may be used as a therapeutic strategy in retinal degeneration either alone or in combination with accepted treatments and deserves further studies in other retinal degeneration models as well as in human retinal diseases.
Data Availability Statement
The original contributions presented in the study are included in the article/Supplementary Material, further inquiries can be directed to the corresponding author.
Ethics Statement
The animal study was reviewed and approved by the Institutional Committee for the Use and Care of Laboratory Animals of the Facultad de Medicina, Universidad de Buenos Aires [CICUAL, Res. (CD) 3130/2017].
Author Contributions
MS and IL performed IHC, TUNEL, WB, and qRT-PCR. EL, MB, and MR-F collaborated in IHC and WB. CFL, EG, LC, and AB collaborated in the treatment with cannabinoid drugs, interpretation of results and manuscript. AM and JL-C designed experiments, interpreted results, directed the research groups and wrote the manuscript.
Funding
This work was supported with grants of the University of Buenos Aires (UBACYT 2014-17/20020130100675BA and 2018/20020170100493BA). IL is supported by a Miguel Servet contract (CP15/00198) from the Instituto de Salud Carlos III-FEDER (Fondo Europeo de Desarrollo Regional, a way to build Europe) and by Fundación Rioja Salud (FRS). AM is supported by Fundación Rioja Salud.
Conflict of Interest
The authors declare that the research was conducted in the absence of any commercial or financial relationships that could be construed as a potential conflict of interest.
Supplementary Material
The Supplementary Material for this article can be found online at: https://www.frontierspartnerships.org/articles/10.3389/adar.2022.10734/full#supplementary-material
References
1. Bisogno, T, and Di Marzo, V. Short-and Long-Term Plasticity of the Endocannabinoid System in Neuropsychiatric and Neurological Disorders. Pharmacol Res (2007) 56(5):428–42. doi:10.1016/j.phrs.2007.09.002
2. Campos, AC, Paraíso-Luna, J, Fogaça, MV, Guimarães, FS, and Galve-Roperh, I. Cannabinoids as Regulators of Neural Development and Adult Neurogenesis. In: Lipidomics of Stem Cells. New York: Humana Press (2017). p. 117–36.
3. Castillo, PE, Younts, TJ, Chávez, AE, and Hashimotodani, Y. Endocannabinoid Signaling and Synaptic Function. Neuron (2012) 76(1):70–81. doi:10.1016/j.neuron.2012.09.020
4. Xu, JY, and Chen, C. Endocannabinoids in Synaptic Plasticity and Neuroprotection. Neuroscientist (2015) 21(2):152–68. doi:10.1177/1073858414524632
5. Basavarajappa, BS, Shivakumar, M, Joshi, V, and Subbanna, S. Endocannabinoid System in Neurodegenerative Disorders. J Neurochem (2017) 142(5):624–48. doi:10.1111/jnc.14098
6. Onaivi, E, Ishiguro, I, Gong, J, Patel, S, Perchuk, A, Meozzi, PA, et al. Discovery of the Presence and Functional Expression of Cannabinoid CB2 Receptors in Brain. Ann N Y Acad Sci (2006) 1074514–536. doi:10.1196/annals.1369.052
7. Brusco, A, Tagliaferro, P, Saez, T, and Onaivi, ES. Postsynaptic Localization of CB2 Cannabinoid Receptors in the Rat hippocampus. Synapse (2008) 62:944–9. doi:10.1002/syn.20569
8. López, EM, Tagliaferro, P, Onaivi, ES, and López-Costa, JJ. Distribution of CB2 Cannabinoid Receptor in Adult Rat Retina. Synapse (2011) 65(5):388–92. doi:10.1002/syn.20856
9. Burstein, S. PPAR-Gamma: a Nuclear Receptor with Affinity for Cannabinoids. Life Sci (2005) 77(14):1674–84. doi:10.1016/j.lfs.2005.05.039
10. Yazulla, S, Studholme, KM, Mc Intosh, HH, and Deutsch, DG. Immunocytochemical Localization of Cannabinoid CB1 Receptor and Fatty Acid Amide Hydrolase in Rat Retina. J Comp Neurol (1999) 41580–90. doi:10.1002/(sici)1096-9861(19991206)415:1<80::aid-cne6>3.0.co;2-h
11. Bouskila, J, Burke, MW, Zabouri, N, Casanova, C, Ptito, M, and Bouchard, JF. Expression and Localization of the Cannabinoid Receptor Type 1 and the Enzyme Fatty Acid Amide Hydrolase in the Retina of Vervet Monkeys. Neuroscience (2012) 202117–130. doi:10.1016/j.neuroscience.2011.11.041
12. Porcella, A, Maxia, C, Gessa, GL, and Pani, L. The Human Eye Expresses High Levels of CB1 Cannabinoid Receptor mRNA and Protein.. Eur J Neurosci (2000) 12(3):1123–7. doi:10.1046/j.1460-9568.2000.01027.x
13. Lu, Q, Straiker, A, Lu, Q, and Maguire, G. Expression of CB2 Cannabinoid Receptor mRNA in Adult Rat Retina. Vis Neurosci (2000) 17(1):91–5. doi:10.1017/s0952523800171093
14. Cécyre, B, Zabouri, N, Huppé-Gourgues, F, Bouchard, J-F, and Casanova, C. Roles of Cannabinoid Receptors Type 1 and 2 on the Retinal Function of Adult Mice. Invest Ophthalmol Vis Sci (2013) 54(13):8079–90. doi:10.1167/iovs.13-12514
15. Galve-Roperh, I, Aguado, T, Palazuelos, J, and Guzmán, M. Mechanisms of Control of Neuron Survival by the Endocannabinoid System. Curr Pharm Des (2008) 14(23):2279–88. doi:10.2174/138161208785740117
16. Matias, I, Wang, JW, Moriello, AS, Nieves, A, Woodward, DF, and Di Marzo, V. Changes in Endocannabinoid and Palmitoylethanolamide Levels in Eye Tissues of Patients with Diabetic Retinopathy and Age-Related Macular Degeneration. Prostaglandins Leukot Essent Fatty Acids (2006) 75(6):413–8. doi:10.1016/j.plefa.2006.08.002
17. El-Remessy, AB, Al-Shabrawey, M, Khalifa, Y, Tsai, N-T, Caldwell, RB, and Liou, GI. Neuroprotective and Blood-Retinal Barrier-Preserving Effects of Cannabidiol in Experimental Diabetes. Am J Pathol (2006) 168:235–44. doi:10.2353/ajpath.2006.050500
18. El-Remessy, AB, Khalil, IE, Matragoon, S, Abou-Mohamed, G, Tsai, N-T, Roon, P, et al. Neuroprotective Effect of (-) Delta9-Tetrahydrocannabinol and Cannabidiol in N-Methyl-D-Aspartate-Induced Retinal Neurotoxicity: Involvement of Peroxynitrite. Am J Pathol (2003) 163(5):1997. doi:10.1016/s0002-9440(10)63558-4
19. Hosseini, A, Lattanzio, FA, Williams, PB, Tibbs, D, Samudre, SS, and Allen, RC. Chronic Topical Administration of WIN-55-212-2 Maintains a Reduction in IOP in a Rat Glaucoma Model without Adverse Effects. Exp Eye Res (2006) 82(5):753–9. doi:10.1016/j.exer.2005.09.017
20. Nucci, C, Gasperi, V, Tartaglione, R, Cerulli, A, Terrinoni, A, Bari, M, et al. Involvement of the Endocannabinoid System in Retinal Damage after High Intraocular Pressure-Induced Ischemia in Rats. Invest Ophthalmol Vis Sci (2007) 48(7):2997–3004. doi:10.1167/iovs.06-1355
21. Crandall, J, Matragoon, S, Khalifa, YM, Borlongan, C, Tsai, N-T, Caldwell, RB, et al. Neuroprotective and Intraocular Pressure-Lowering Effects of (-)Delta9-tetrahydrocannabinol in a Rat Model of Glaucoma. Ophthalmic Res (2007) 39(2):69–75. doi:10.1159/000099240
22. Lax, P, Esquiva, G, Altavilla, C, and Cuenca, N. Neuroprotective Effects of the Cannabinoid Agonist HU210 on Retinal Degeneration. Exp Eye Res (2014) 120:175–85. doi:10.1016/j.exer.2014.01.019
23. Abler, AS, Chang, CJ, Ful, J, Tso, MO, and Lam, TT. Photic Injury Triggers Apoptosis of Photoreceptor Cells. Res Commun Mol Pathol Pharmacol (1996) 92:177–89.
24. López, EM, Julián, LK, Capani, F, Cymeryng, CB, Coirini, H, and López-Costa, JJ. Endogenous Glucocorticoids Participate in Retinal Degeneration during Continuous Illumination. Int J Neurosci (2008) 118:1725–47. doi:10.1080/00207450802174449
25. Marti, A, Hafezi, F, Lansel, N, Hegi, ME, Wenzel, A, Grimm, C, et al. Light-induced Cell Death of Retinal Photoreceptors in the Absence of P53. Invest Ophthalmol Vis Sci (1998) 39:846–9.
26. Mittag, TW, Bayer, AU, and La Vail, MM. Light-induced Retinal Damage in Mice Carrying a Mutated SOD I Gene. Exp Eye Res (1999) 69:677–83. doi:10.1006/exer.1999.0748
27. Piehl, L, Capani, F, Facorro, G, López, EM, Rubin de Celis, E, Pustovrh, C, et al. Nitric Oxide Increases in the Rat Retina after Continuous Illumination. Brain Res (2007) 1156112–9. doi:10.1016/j.brainres.2007.04.030
28. Remé, CE, Grimm, C, Hafezi, F, Iseli, HP, and Wenzel, A. Why Study Rod Cell Death in Retinal Degenerations and How? Doc Ophthalmol (2003) 106:25–9. doi:10.1023/a:1022423724376
29. Chader, GJ. Animal Models in Research on Retinal Degenerations: Past Progress and Future hope. Vis Res. (2002) 42:393–9. doi:10.1016/s0042-6989(01)00212-7
30. Gorn, RA, and Kuwabara, T. Retinal Damage by Visible Light. A Physiologic Study. Arch Ophthalmol (1967) 77:115–8. doi:10.1001/archopht.1967.00980020117025
31. Hafezi, F, Marti, A, Munz, K, and Remé, CE. Light-induced Apoptosis: Differential Timing in the Retina and Pigment Epithelium. Exp Eye Res (1997) 64:963–70. doi:10.1006/exer.1997.0288
32. Noell, WK, Walker, VS, Kang, BS, and Berman, S. Retinal Damage by Light in Rats. Invest Ophthalmol (1966) 5:450–73.
33. Pecci Saavedra, J, Pellegrino de Iraldi, A, and Pellegrino de IrAldi, A. Retinal Alterations Induced by Continuous Light in Immature Rats. I. Fine Structure and Electroretinography. Cell Tissue Res. (1976) 166:201–11. doi:10.1007/BF00227041
34. Margalit, E, and Sadda, SR. Retinal and Optic Nerve Diseases. Artif Organs (2003) 27:963–74. doi:10.1046/j.1525-1594.2003.07304.x
35. Ambati, J, Ambati, BK, Yoo, SH, Ianchulev, S, and Adamis, AP. Age-related Macular Degeneration: Etiology, Pathogenesis, and Therapeutic Strategies. Surv Ophthalmol (2003) 48:257–93. doi:10.1016/s0039-6257(03)00030-4
36. Pennington, KL, and DeAngelis, MM. Epidemiology of Age-Related Macular Degeneration (AMD): Associations with Cardiovascular Disease Phenotypes and Lipid Factors. Eye Vis (2016) 3:34. doi:10.1186/s40662-016-0063-5
37. Soliño, M, López, EM, Rey-Funes, M, Loidl, CF, Larrayoz, IM, Martínez, A, et al. Adenosine A1 Receptor: a Neuroprotective Target in Light Induced Retinal Degeneration. PLoS One (2018) 13:e0198838. doi:10.1371/journal.pone.0198838
38. Soliño, M, Larrayoz, IM, López, EM, Rey-Funes, M, Bareiro, M, Loidl CF, , et al. Adenosine A2A Receptor: A New Neuroprotective Target in Light-Induced Retinal Degeneration. Front Pharmacol (2022) 13:840134. doi:10.3389/fphar.2022.840134
39. Mulè, F, Amato, A, Baldassano, S, and Serio, R. Involvement of CB1 and CB2 Receptors in the Modulation of Cholinergic Neurotransmission in Mouse Gastric Preparations. Pharmacol Res (2007) 56(3):185–92. doi:10.1016/j.phrs.2007.06.002
40. Hancock, MB. Visualization of Peptide-Immunoreactive Processes on Serotonin-Immunoreactive Cells Using Two-Color Immunoperoxidase Staining. J Histochem Cytochem (1984) 32:311–4. doi:10.1177/32.3.6198359
41. Kawajiri, K, and Fujii-Kuriyama, Y. Cytochrome P450 Gene Regulation and Physiological Functions Mediated by the Aryl Hydrocarbon Receptor. Arch Biochem Biophys (2007) 464207–212. doi:10.1016/j.abb.2007.03.038
42. Machado, FS, Johndrow, JE, Esper, L, Dias, A, Bafica, A, Serhan, CN, et al. Anti-inflammatory Actions of Lipoxin A4 and Aspirin-Triggered Lipoxin Are SOCS-2-dependent. Nat Med (2006) 12:330–4. doi:10.1038/nm1355
43. Basrai, HS, and Turnley, AM. The Suppressor of Cytokine Signalling 2 (SOCS2), Traumatic Brain Injury and Microglial/macrophage Regulation. Neural Regen Res (2016) 11(9):1405–6. doi:10.4103/1673-5374.191206
44. Nagayama, T, Sinor, AD, Chen, J, Grahan, SH, Jin, K, Greenberg, DA, et al. Cannabinoids and Neuroprotection in Global and Focal Cerebral Ischemia and in Neuronal Cultures. J Neurosci (1999) 19(8):2987–95. doi:10.1523/jneurosci.19-08-02987.1999
45. Parmentier-Batteur, S, Jin, K, Mao, XO, Xie, L, and Greenberg, DA. Increased Severity of Stroke in CB1 Cannabinoid Receptor Knock-Out Mice. J Neurosci (2002) 22(22):9771–5. doi:10.1523/jneurosci.22-22-09771.2002
46. Liu, Q, Bhat, M, Bowen, WD, and Cheng, J. Signaling Pathways from Cannabinoid Receptor-1 Activation to Inhibition of N-Methyl-D-Aspartic Acid Mediated Calcium Influx and Neurotoxicity in Dorsal Root Ganglion Neurons. J Pharmacol Exp Ther (2009) 331:1062–70. doi:10.1124/jpet.109.156216
47. Galve-Roperh, I, Rueda, D, Gómez del Pulgar, T, Velasco, G, and Guzmán, M. Mechanism of Extracellular Signal-Regulated Kinase Activation by the CB(1) Cannabinoid Receptor. Mol Pharmacol (2002) 62:1385–92. doi:10.1124/mol.62.6.1385
48. Esposito, G, De Filippis, D, Carnuccio, R, Izzo, AA, and Iuvone, T. The Marijuana Component Cannabidiol Inhibits Beta-Amyloid-Induced Tau Protein Hyperphosphorylation through Wnt/beta-Catenin Pathway rescue in PC12 Cells. J Mol Med (2006) 84:253–8. doi:10.1007/s00109-005-0025-1
49. Mackie, K, Lai, Y, Westenbroek, R, and Mitchell, R. Cannabinoids Activate an Inwardly Rectifying Potassium Conductance and Inhibit Q-type Calcium Currents in Att20 Cells Transfected with Rat Brain Cannabinoid Receptor. J Neurosci (1995) 15:6552–61. doi:10.1523/jneurosci.15-10-06552.1995
50. Organisciak, DT, Darrow, RM, Barsalou, L, Darrow, RA, Kutty, RK, Kutty, G, et al. Light History and Age-Related Changes in Retinal Light Damage. Invest Ophthalmol Vis Sci (1998) 39:1107–16.
51. Wu, J, Gorman, A, Zhou, X, Sandra, C, and Chen, E. Involvement of Caspase-3 in Photoreceptor Cell Apoptosis Induced by In Vivo Blue Light Exposure. Invest Ophthalmol Vis Sci (2002) 43:3349–54.
52. Grimm, C, Wenzel, A, Hafezi, F, and Remé, CE. Gene Expression in the Mouse Retina: The Effect of Damaging Light. Mol Vis (2000) 6:252–60.
53. Abbler, AS, Chang, CJ, Full, J, Tso, MO, and Lam, TT. Photic Injury Triggers Apoptosis of Photoreceptor Cells. Res Commun Mol Pathol Pharmacol (1996) 92177–189.
54. Virmani, MA, and Cirulli, M. The Role of L-Carnitine in Mitochondria, Prevention of Metabolic Inflexibility and Disease Initiation. Int J Mol Sci (2022) 23:2717. doi:10.3390/ijms23052717
55. Slusar, JE, Cairns, EA, Szczesniak, AM, Bradshaw, HB, Di Polo, A, and Kelly, MEM. The Fatty Acid Amide Hydrolase Inhibitor, URB597, Promotes Retinal Ganglion Cell Neuroprotection in a Rat Model of Optic Nerve Axotomy. Neuropharmacology (2013) 72:116–25. doi:10.1016/j.neuropharm.2013.04.018
56. Zong, Y, Zhou, X, Cheng, J, Yu, J, Wu, J, and Jiang, C. Cannabinoids Regulate the Diameter of Pericyte-Containing Retinal Capillaries in Rats. Cell. Physiol. Biochem. (2018) 43(5):2088–101. doi:10.1159/000484193
57. Chen, Y, Luo, X, Liu, S, and Shen, Y. Neuroprotective Effect of Cannabinoid Receptor 1 Antagonist in the MNU-Induced Retinal Degeneration Model. Exp Eye Res (2018) 167:145–51. doi:10.1016/j.exer.2017.11.001
58. El-Remessy, AB, Rajesh, M, Mukhopadhyay, P, Horvárth, B, Patel, V, Al-Gayyar, MMH, et al. Cannabinoid 1 Receptor Activation Contributes to Vascular Inflammation and Cell Death in a Mouse Model of Diabetic Retinopathy and a Human Retinal Cell Line. Diabetologia (2011) 54(6):1567–78. doi:10.1007/s00125-011-2061-4
59. Maccarone, R, Rapino, C, Zerti, D, di Tommaso, M, Battista, N, Di Marco, S, et al. Modulation of Type-1 and Type-2 Cannabinoid Receptors by Saffron in a Rat Model of Retinal Neurodegeneration. Plos One (2016) 11:e0166827. doi:10.1371/journal.pone.0166827
60. Pacher, P, Batkai, S, and Kunos, G. The Endocannabinoid System as an Emerging Target of Pharmacotherapy. Pharmacol Rev (2006) 58(3):389–462. doi:10.1124/pr.58.3.2
Keywords: cannabinoid, CB1, degeneration, neuroprotection, retina
Citation: Soliño M, Larrayoz IM, López EM, Rey-Funes M, Bareiro M, Loidl CF, Girardi E, Caltana L, Brusco A, Martínez A and López-Costa JJ (2022) CB1 Cannabinoid Receptor is a Target for Neuroprotection in Light Induced Retinal Degeneration. Adv. Drug. Alco. Res. 2:10734. doi: 10.3389/adar.2022.10734
Received: 29 June 2022; Accepted: 23 August 2022;
Published: 13 September 2022.
Edited by:
Emmanuel Onaivi, William Paterson University, United StatesReviewed by:
Rita Maccarone, University of L’Aquila, ItalyMohamed Ashraf Virmani, Alfasigma Nederland B.V., Netherlands
Copyright © 2022 Soliño, Larrayoz, López, Rey-Funes, Bareiro, Loidl, Girardi, Caltana, Brusco, Martínez and López-Costa. This is an open-access article distributed under the terms of the Creative Commons Attribution License (CC BY). The use, distribution or reproduction in other forums is permitted, provided the original author(s) and the copyright owner(s) are credited and that the original publication in this journal is cited, in accordance with accepted academic practice. No use, distribution or reproduction is permitted which does not comply with these terms.
*Correspondence: Juan José López-Costa, ampsb3BlekBmbWVkLnViYS5hcg==
†Deceased 2022
‡These authors have contributed equally to this work and share first authorship
§These authors share last authorship