- 1Department of Pharmaceutical Sciences, University of Connecticut, Storrs, CT, United States
- 2Connecticut Institute for the Brain and Cognitive Sciences (CT IBACS), Storrs, CT, United States
Although noncoding RNAs (ncRNAs) have been shown to regulate maladaptive neuroadaptations that drive compulsive drug use, ncRNA-targeting therapeutics for substance use disorder (SUD) have yet to be clinically tested. Recent advances in RNA-based drugs have improved many therapeutic issues related to immune response, specificity, and delivery, leading to multiple successful clinical trials for other diseases. As the need for safe and effective treatments for SUD continues to grow, novel nucleic acid-based therapeutics represent an appealing approach to target ncRNA mechanisms in SUD. Here, we review ncRNA processes implicated in SUD, discuss recent therapeutic approaches for targeting ncRNAs, and highlight potential opportunities and challenges of ncRNA-targeting therapeutics for SUD.
Introduction
Substance use disorder (SUD) continues to be a worldwide public health crisis (1). Although many of the underlying mechanisms that drive compulsive drug use have been elucidated, the number of pharmacological agents that are approved to treat SUD remains stagnant (2). Current pharmacotherapies for SUD largely consist of small molecule modulation of neurotransmitter receptor activity (2). While these treatments have shown some clinical success, many promising therapeutic opportunities will likely be missed if this narrow focus continues. Thus, to move the field forward and to improve patient outcomes, novel pharmacological interventions for SUD are greatly needed.
As only 1%–2% of the human genome encodes for protein (3, 4) and many proteins lack druggable sites for small molecules (5), researchers are turning to nucleic acid-based treatments to target previously undruggable mechanisms. The recent progress in nucleic acid chemistry, bioinformatic approaches, and delivery systems has dramatically improved several issues associated with stability, specificity, and tolerability of RNA-targeting drugs (6). These advancements have resulted in successful clinical trials and recent approvals of nucleic acid-based therapeutics by the Food and Drug Administration (FDA) and the European Medicines Agency (EMA) for various disorders (7, 8). Additional factors contributing to the rising interest and growth in nucleic acid-based therapeutics include rationale design, rapid optimization and adaptability to evolving targets, high selectivity, and potentially longer half-life leading to infrequent administration (7, 8). While many of these initial therapies aimed to modulate protein-coding transcripts, more recently, there has been a rising interest in developing nucleic acid-based drugs that target noncoding RNAs (ncRNAs), given their significant roles in cell type-specific biological processes in both health and disease (9).
In animal models of SUD, several ncRNAs have been shown to play functional roles in drug-seeking behaviors (10), and in humans, many genetic variants linked to SUD are located within noncoding regions of the genome (11). Thus, as the number of putative ncRNA targets in SUD continues to grow, nucleic acid-based therapeutics will likely be required to modulate these novel mechanisms. In this review, we describe different ncRNA classes involved in SUD, provide an overview of the modalities used to manipulate ncRNAs, and highlight ncRNA-based treatment strategies for SUD. We also discuss the ongoing challenges of ncRNA targeting and provide future perspectives for ncRNA-based therapeutics in SUD.
Noncoding RNAs in SUD
In humans and other primates, ncRNA expansion has fostered the intricate regulatory network required for brain evolution and cognitive advancement (12). ncRNAs are abundantly expressed in the central nervous system (CNS) where many are transcribed in a cell type-specific manner (13). In neuropsychiatric disorders, including SUD, changes in brain ncRNA expression have been associated with disease pathophysiology (13, 14), and several ncRNAs have been functionally examined in CNS disease models (15–17). In SUD, most of the research has focused on 3 classes of ncRNAs: microRNAs (miRs), long noncoding RNAs (lncRNAs), and more recently circular RNAs (circRNAs) (Table 1). In this section, we briefly review the mechanistic roles of miRs, lncRNAs, and circRNAs, and highlight potential therapeutic ncRNA targets in SUD.
MicroRNAs
MicroRNAs are a class of small noncoding RNAs with a highly conserved single-stranded sequence of approximately 22 nucleotides (36). Initially, miRs are transcribed into longer primary transcripts, called pri-miRs. The pri-miR is then cleaved by Drosha in the nucleus to produce the precursor miR (pre-miR) before being processed by Dicer in the cytosol to yield the mature miR. The mature miR is then loaded into the RNA-induced silencing complex (RISC) where it hybridizes to the three prime untranslated region (3′-UTR) of target mRNAs to mediate translational inhibition, cleavage, or degradation (36). With the ability to modulate 20%–50% of protein-coding genes, miRs are considered master regulators of many cellular activities (37–39). Notably, miRs play essential roles in brain development and neuroplasticity, and their dysregulation has been linked to the pathophysiology of most neuropsychiatric disorders (40–42).
In preclinical and clinical SUD studies, many miRs are dysregulated in reward-related brain regions following cocaine (25, 26, 43–48), amphetamine (49–51), methamphetamine (28–30, 52–57), nicotine (34, 58–63), opioid (31, 32, 64–71), and alcohol use (19, 20, 22, 72–83). SUD-associated miRs and their underlying mechanisms have been thoroughly reviewed elsewhere (14, 84). Of the miRs correlated with drug use, several have been shown to regulate the expression of known SUD targets that play important roles in maladaptive neuroplasticity and drug-seeking behaviors (e.g., BDNF, CREB, MeCP2, CaMKIIa) (14). In particular, miR-212, miR-132, miR-181, miR-9, and let-7 may be of interest for clinical targeting as altered expression of these miRs has been observed across multiple drugs of abuse in human and animal samples (14). In addition to miR activity in the brain, miR levels in SUD patient blood samples have been correlated with drug history and relapse (23, 85–94). Thus, circulating miRs may be a useful auxiliary measurement for diagnosis and treatment.
While there have been no clinical trials using miR-targeting therapeutics in SUD patients, several miRs have been explored functionally in preclinical SUD models (Table 1). For example, viral-mediated overexpression of miR-124a in the dorsolateral striatum enhanced alcohol-induced conditioned place preference (CPP) and increased alcohol intake, while silencing its expression attenuated CPP and alcohol consumption (20). In cocaine CPP experiments, overexpression of miR-124 and let-7d in the nucleus accumbens (NAc) attenuated cocaine CPP, whereas miR-181a overexpression enhanced CPP (95). The opposite effect on cocaine CPP was observed following knockdown of miR-124, let-7d, and miR-181a in the NAc. In self-administration studies, overexpression of miR-212 in the dorsal striatum attenuated compulsive cocaine intake in the extended-access self-administration procedure (25). Consistent with these observations, reduced levels of miR-212 in the striatum were associated with cocaine intake in addiction-prone but not addiction-resistant rats (96). In opioid self-administration experiments, overexpression of miR-132 in dentate gyrus increased morphine-seeking behaviors (32), while in a different study, overexpression of miR-9 in the NAc increased oxycodone intake and reduced inter-infusion interval (31). Overall, these results indicate that miRs are important therapeutic targets in SUD.
Long noncoding RNAs
Long noncoding RNAs (lncRNAs) are a diverse class of RNA molecules that are greater than 200 nucleotides in length and are generally classified based on their genomic location or function (e.g., intronic, intergenic, antisense, and enhancer) (97). Many lncRNAs are expressed in a cell-type and tissue-specific manner and play important regulatory roles in cells by acting as decoy, guide, scaffold, and/or signaling molecules (97, 98). For example, lncRNAs have been shown to mediate gene-specific epigenetic modifications by recruiting chromatin-modifying complexes to their targets (99, 100). At the post-transcriptional level, lncRNAs also fine-tune mRNA splicing, stability, and translation (97). In the mammalian nervous systems, many lncRNAs are highly enriched within the brain and play essential roles in the complex spatio-temporal gene expression mechanisms during brain development and neuroplasticity (98). Consequently, altered lncRNA expression is inherent to several brain diseases, including SUD (10).
One of the first attempts to examine a role for lncRNAs in SUD was made by analyzing lncRNA expression in the NAc of post-mortem heroin- and cocaine-using subjects (101). Relative to drug-free controls, an upregulation of MIAT, NEAT1, MALAT1, and MEG3 lncRNAs was observed in the NAc of heroin-using subjects, and MIAT, MALAT1, MEG3, and EMX2OS upregulation was observed in the NAc of cocaine-using subjects. These well-studied lncRNAs contribute to various cellular processes, including GABA neuron neurogenesis, synapse formation, and cAMP signaling (102–104). In rodent studies, transcriptional profiling of lncRNAs in the NAc of methamphetamine-treated mice revealed thousands of lncRNAs that were altered, mostly downregulated by methamphetamine (105). Further bioinformatic analysis revealed that several of these lncRNAs act as potential cis- or trans-regulators of protein-coding genes involved in reward and addiction pathways. In other experiments, lncRNAs, including H19, Mirg, BC1, Lrap, and Gas5 have also been linked to SUDs (27, 106–110). Although most SUD-related lncRNA experiments have been limited to correlational data, Xu et al. recently revealed a functional role for the lncRNA Gas5 in SUD models (111). In these studies, cocaine exposure (intraperitoneal injections and self-administration) reduced Gas5 expression in the NAc, and in behavioral experiments, viral-mediated overexpression of Gas5 in the NAc attenuated cocaine CPP and self-administration. At the transcriptomic level, Gas5-regulated gene expression patterns overlapped significantly with genes altered by cocaine exposure, an indication that Gas5 regulates cocaine-induced transcriptional responses.
Natural antisense transcripts (NATs) are a class of lncRNAs that have also been implicated in SUD (112). NATs are transcribed from the opposite (antisense) strand of a coding gene and partially or completely overlap with the body, promoter, or enhancer region of the coding gene. Many genes involved in drug-induced neuroplasticity contain NATs (113), and the expression of multiple NATs such as Bdnf-AS, Homer1-AS, Traf3ip2-AS1, and Prkcq-AS1 is altered by drugs of abuse (35, 113, 114). Therefore, NAT inhibition could be a particularly useful approach to increase the expression of SUD-related protein-coding genes. As a proof of concept, researchers have found that knockdown of Bdnf-AS in the infralimbic cortex via antisense oligonucleotides attenuated nicotine self-administration (115), and in other experiments, siRNA-mediated silencing of Bdnf-AS attenuated ketamine-induced neurotoxicity (116). Thus, with their high target specificity and their emerging roles in drug-seeking behaviors, lncRNAs are promising therapeutic targets for SUD.
Circular RNAs
Circular RNAs (circRNAs) are single-stranded noncoding RNA molecules produced from pre-mRNAs by a non-canonical splicing process called back-splicing, resulting in covalently closed RNA loops. Approximately 20% of mammalian genes express circRNAs, and while these ncRNAs are present in various organs, their enriched expression in the brain makes them an appealing target for the treatment of neuropsychiatric disorders (117, 118). circRNAs play important roles as transcriptional, post-transcriptional, and/or translational regulators through various mechanisms, most notably as a sponge for miRs (119). Compared to linear RNAs, circRNAs are highly stable (120), and thus may also mediate long-term effects in several disease states.
In several recent papers, a role for circRNAs in SUD has been explored. For example, RNA-sequencing analysis of post-mortem human NAc samples identified several circRNA–miR interactions that were associated with alcohol dependence (121), and in rodent studies, prenatal alcohol exposure was shown to alter the expression of brain circRNAs in a sex-specific manner (122). circRNAs are also dysregulated by opioids (24, 33, 123). In particular, CircTmeff-1, a sponge of miR-541-5p and miR-6934-3p, was observed to be functionally important for morphine CPP (24) and more recently for the reconsolidation of cocaine CPP (124). In other psychostimulant studies, 90 mouse striatal circRNAs were differentially expressed following cocaine self-administration (125), and 41 differentially expressed circRNAs were discovered in the dorsolateral prefrontal cortex of post-mortem human subjects with cocaine use disorder (126). Finally, in methamphetamine-induced neurotoxicity models, numerous circRNAs were significantly altered following methamphetamine treatment (127), and knockdown of circHomer1 alleviated methamphetamine-induced toxicity (128). Together, these initial experiments indicate an important and emerging role for circRNAs in drug-induced neuroadaptations.
Categories of ncRNA-targeting drugs
Due to significant improvements in safety, selectivity and delivery, RNA-based pharmaceuticals have received considerable attention and 14 RNA-based drugs have received FDA or EMA approval since 2015. See reference (129) for a comprehensive review of current FDA-approved RNA therapeutics. In addition to using nucleic acids to target RNAs, researchers have also developed small molecules that target RNA transcripts, termed small molecules interacting with RNA (SMIRNAs) (130). While the initial strategies to target RNAs focused on coding genes, many preclinical and clinical studies are now using similar approaches to target ncRNAs (Figure 1). In this section, we will briefly review the major categories of ncRNA-targeting drugs and highlight potential therapeutic opportunities for each platform in the context of SUD.
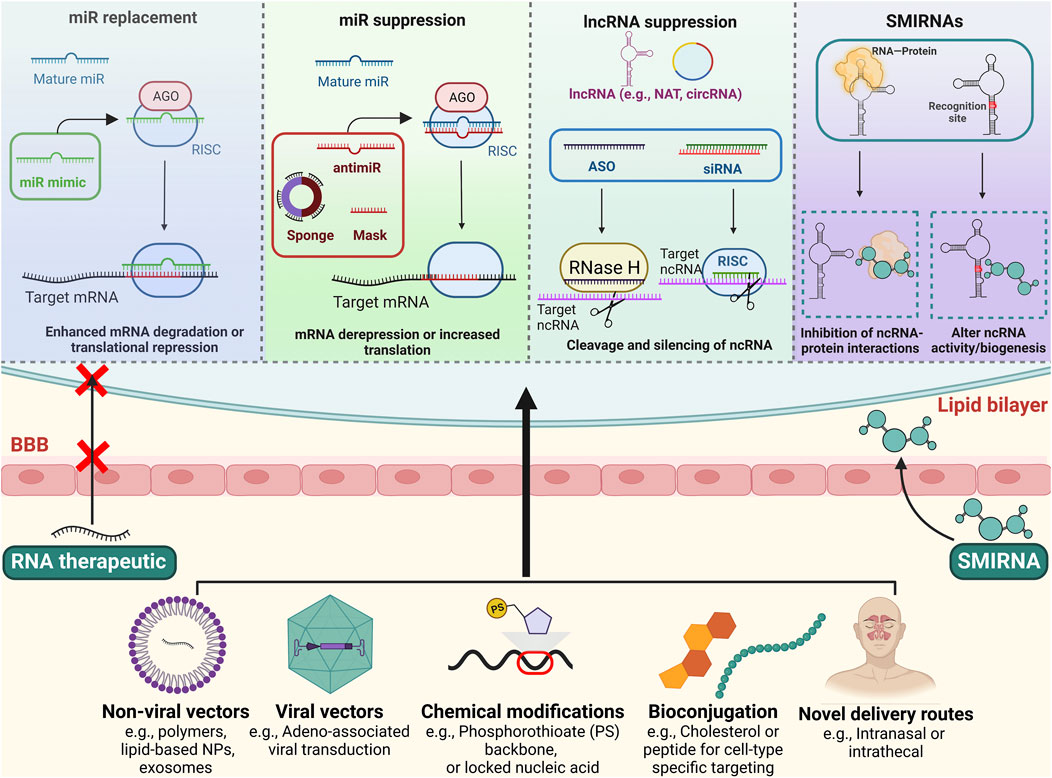
FIGURE 1. Schematic overview of RNA-targeting therapeutics and strategies to improve CNS delivery. Top: Multiple approaches exist for targeting ncRNAs. For miR replacement, miR mimics are used to imitate endogenous miRs activity, whereas antimiRs, miR masks and sponges inhibit endogenous miR activity. lncRNAs can be targeted with ASOs and siRNAs, leading to their degradation and silencing. SMIRNAs are small molecules that directly bind to ncRNAs or interfere with ncRNA-protein interactions. Bottom: Nanoparticles, viral vectors, chemical modifications, and/or bioconjugations can facilitate stability, cellular uptake, and brain delivery of the ncRNA therapeutics. Novel delivery routes, such as intranasal and intrathecal administration, may also promote CNS delivery and limit systemic toxicities. Some drug-like SMIRNAs are able to cross the blood-brain barrier without a delivery system via passive diffusion. Figure created using BioRender.
Antisense oligonucleotides
Antisense oligonucleotides (ASOs) are small, synthetic single-stranded nucleic acid molecules that hybridize with the target RNA to alter splicing or translation via steric block or RNA degradation (7). The smaller size and stringent binding specificity give ASOs a therapeutic advantage in CNS-related diseases compared to other nucleic acid drugs (Table 2). Indeed, several ASOs that are in clinical trials are being used to treat CNS-related diseases (131). Also, unlike siRNAs, ASOs are able to increase target protein expression by promoting alternative splicing, a strategy that is used clinically for Duchenne muscular dystrophy and spinal muscular atrophy (132).
Unmodified or naked ASOs display significant immunogenicity, low stability, and are rapidly cleared from circulation (133). Thus, chemical modifications are necessary to improve pharmacokinetics and pharmacodynamics of ASO pharmaceuticals (for a comprehensive review see (134)). Common ASO modifications include substitution of a phosphorothioate (PS) backbone and sugar moiety modifications at 2′ position (e.g., 2′-O-methyl, locked nucleic acid, LNA) (134). Though each type of chemically modified ASOs has advantages and disadvantages, in general, these modifications increase safety, stability, and affinity while reducing the need for delivery systems. However, because most ASOs and other nucleic acids are unable to cross the blood-brain barrier, intrathecal or intranasal administration is typically required to target the CNS (135). Currently, there is at least one ncRNA-targeting ASO undergoing clinical testing for Angelman syndrome (NCT05127226) after successful in vitro and in vivo investigations (136). A few SUD-associated lncRNAs (e.g., MALAT1, MIAT, and BDNF-AS) have been successfully targeted using ASOs in other preclinical disease models (137–141), but additional work is needed to determine if these or other ncRNA-targeting ASO formulations are effective in SUD models.
siRNAs
SiRNAs are short double-stranded RNAs that attach to RISC, unfold, and form Watson-Crick base pairing with the target RNA, leading to argonaute-induced degradation of the transcript (129). Like ASOs, chemical modifications to siRNAs have improved their safety and efficacy (142–144) and currently 5 siRNA-based drugs have received FDA or EMA approval (Patisiran, Givosiran, Lumasiran, Inclisiran, Vutrisiran). However, in contrast to some ASOs, siRNA platforms depend on the intracellular machinery for their effects, which may restrict the type and number of chemical modifications to the siRNA. Also, in some instances, siRNAs are not as effective at targeting nuclear RNAs compared to ASOs (145), and because of their larger size and negative charge, unmodified siRNAs require the use of a delivery agent to enter the cell (Table 2). To combat some of these limitations, researchers have developed siRNA prodrugs (siRibonucleic neutrals, siRNNs) that disguise the siRNAs’ negative charge by replacing phosphodiesters with charge-neutral phosphotriesters (146). These siRNA prodrugs are able to cross the lipid bilayer, and once in the cell, the phosphotriester group is cleaved off by thioesterases, allowing for the knockdown to occur.
While most FDA-approved siRNA drugs target the liver, there has been a growing interest in using novel siRNA formulations to treat CNS-related disorders. For example, Regeneron Pharmaceuticals and Alnylam Pharmaceuticals recently announced a billion-dollar collaboration to develop siRNA-based drugs for CNS indications (147). Further supporting the usefulness of siRNA-based drugs for CNS uses, recent preclinical experiments identified novel, chemically modified siRNAs that exhibited safe, potent, and long-lasting gene silencing in the brain of rodents and nonhuman primates following intrathecal administration (148). Using systemic or direct brain injections, siRNA-targeting of ncRNAs has been achieved in animal models of SUD (124, 149, 150), Parkinson’s disease (151, 152), Alzheimer’s disease (153–155), epilepsy (156, 157) and stroke (158, 159). Thus, with recent FDA approvals, multiple ongoing, late-stage clinical tests, and promising preclinical data, siRNA-based therapeutics appear to have a promising future, but more testing of siRNA formulations for CNS indications is needed.
miR replacement/suppression
MiR targeting has been achieved using RNA interference approaches. For example, miR mimics are modified double-stranded RNA molecules that imitate endogenous miR activity and bind to the 3′UTR region of the target mRNAs (37). This approach leads to a downregulation of the target mRNAs via translational inhibition. On the other hand, antimiRs, miR sponges, and miR masking techniques are used to reduce miR activity. Structurally similar to ASOs, miR inhibitors or antimiRs prevent an endogenous miRs interaction with its target genes. These single-stranded molecules are usually modified using locked nucleic acid, peptide nucleic acids, or cholesterol (i.e., antagomiR) to improve stability, cellular uptake, and in vivo delivery (134, 160). To inhibit a family of miRs, miR sponges, synthetic transcripts that contain various complementary sequences that recognize the seed sequences of multiple miRs, have also been employed in preclinical studies (161–163). Finally, in a technique called miR masking, ASOs bind to 3′UTR sites on a specific mRNA and prevent its interaction with a complementary miR (21) (Figure 1).
In preclinical studies, researchers have demonstrated the effectiveness of antimiRs in animal models of alcohol (19, 164–166), cocaine (25, 124), and opioid (167) use disorders via intrathecal or direct brain injections. In other disease models, SUD-relevant miRs (miR-34, miR-145, miR-212) have been targeted with miR mimics (168–170). Although miR-based therapeutics have yet to be tested clinically in SUD patients, several miR mimic and antimiR formulations are being tested in animals or clinical trials for other diseases (171–175). To move the field of miR-targeted SUD therapeutics forward, researchers are encouraged to identify miRs that drive relapse and craving (rather than acquisition of drug-seeking behaviors) and test clinically relevant miR-targeted formulations in sophisticated SUD models.
Small molecules interacting with RNAs
Emerging research indicates that the three-dimensional structure of RNA, which creates well-defined recognition sites and motifs, can be selectively targeted with small molecules (176). Other than directly binding to specific RNAs (including ncRNAs), SMIRNAs are also able to indirectly affect the RNA functions by interfering with RNA biogenesis or RNA-protein interactions (177–179) (Figure 1). Unlike nucleic acid-based treatments, many SMIRNAs have low molecular weights (usually <1 KDa) and may be administered orally (180), important factors for translational applications (Table 2). However, the likelihood of discovering a small molecule with favorable drug-like characteristics depends on the selected RNA target (181). In other words, the RNA must contain a unique recognition site with considerable structural complexity, differentiating it from other RNAs to avoid non-specific binding and side effects. Also, the abundance of the RNA may influence the efficacy of SMIRNAs (182), a potential issue when targeting very low expressing lncRNAs.
Despite the aforementioned challenges, several SMIRNAs have been identified and validated in preclinical studies (183–189), and in 2020 Risdiplam (an orally available, non-antibiotic SMIRNA) received FDA approval for the treatment of spinal muscular atrophy (190). ncRNAs have also been successfully targeted with SMIRNAs. For example, two studies have identified SMIRNAs for MALAT1 (191, 192), a lncRNA that is altered in the brain of heroin and cocaine users and in rats treated with morphine (101, 193). In other studies, a first-in-class, clinical-stage quinolone compound, ABX464, was found to increase the expression of miR-124, a target that has been well-studied in SUD models (194). This molecule has passed phase I dose safety trial and phase IIa clinical studies, and although ABX464 has been mainly studied in HIV and Ulcerative Colitis, it could also be used to upregulate miR-124 expression in the brain to reduce drug-induced neurobehavioral adaptations (194). NP-C86 is another SMIRNA that stabilizes the lncRNA Gas5 (195), a lncRNA that has been associated to cocaine-seeking behaviors (111). Finally, the let-7 family, miRs with a known link to SUD, are suppressed by RNA-binding proteins called LIN28. Recently, Wang et al. successfully identified six small molecule disruptors of LIN28 and subsequently let-7 suppression (179). Together, these studies indicate that targeting ncRNAs with SMIRNAs is a feasible approach and may have potential utility in SUD.
Delivery systems for ncRNA therapeutics
Despite several advances, treating CNS diseases with nucleic acids-based platforms remains a major challenge due to the blood-brain barrier. Comprised of tight junctions between brain capillary endothelial cells, the blood-brain barrier prevents large molecule therapeutics from entering the brain parenchyma. To circumvent this issue, researchers have developed several RNA delivery systems that are capable of entering the brain via intravenous, intrathecal, or intranasal routes of administration (131, 196–200). Viral vectors and nanoparticle carrier systems are some of the most promising strategies for delivering ncRNA therapeutics to the brain and are discussed below.
Viral vectors
In preclinical studies, viral vectors are widely used to transfer nucleic acids to brain cells with high efficiency (201). The most commonly used viral vectors for delivering nucleic acids are adenovirus, adeno-associated virus (AAV), and lentivirus vectors (202–204). In neuroscience research, AAVs are especially popular as different serotypes allow for transduction of distinct brain cells (205) and projection-specific pathways (206). Another advantage of viral vectors is the ability to target disease-related brain cells, using cell type-specific promoters (207, 208). However, the vast majority of SUD-related studies that have used viral vectors to manipulate ncRNAs have done so by direct brain injections, an approach that may have limited clinical utility. More recently, researchers have developed viral vectors that are capable of targeting the brain via more feasible routes of administration. For example, intrathecal injection of an AAV that expresses an artificial miR resulted in robust gene silencing with no observed side effects in nonhuman primates (209). Using the same approach, a case study in ALS patients also generated promising results (210). In animal models of Huntington’s disease, intravenous injection of a novel AAV encoding an artificial miR that targets the huntingtin (HTT) gene yielded extensive knockdown of HTT across multiple brain regions with the highest transduction observed in the striatum (199). Several other studies have also explored viral-mediated CNS delivery of ncRNAs via intrathecal or intravenous routes of administration (211–215) and multiple clinical trials using AAVs in Parkinson’s disease, Alzheimer’s disease, Batten disease, and Canavan disease patients have been conducted or in progress (216). In summary, nonpathogenic viral vectors offer a powerful option for ncRNA-targeted brain delivery and should be further pursued in SUD patients.
Nanoparticles
Nanoparticle-mediated delivery of ncRNA therapeutics is a promising approach for the treatment of SUD (217). Nanoparticles have several appealing properties including, tunable release rate, biocompatibility, limited toxicity, brain penetrating capabilities, and adjustable surface modifications for cell type-specific delivery (218). Many different classes of nanocarriers have been successfully tested in CNS disease models, including polymeric, inorganic, exosome, and lipid-based nanoparticles (219–229), and as an indication of their safety and efficacy across multiple disease states, several nanoparticle formulations have received FDA approval, including the recent approvals of the Pfizer-BioNTech and Moderna COVID-19 vaccines (both use lipid nanoparticles for mRNA delivery) and the siRNA drug Patisiran (230).
Although nanoparticle-mediated brain delivery via systemic administration remains an ongoing challenge, miR mimic and antimiR encapsulated nanoparticles have successfully targeted the brain in multiple CNS disease models following systemic administration (231–233). For example, intranasal delivery of extracellular vesicles loaded with miR-124 to cocaine-treated mice resulted in successful brain uptake and abrogation of inflammatory markers (234). A more recent strategy for the delivery of nucleic acids to the brain is to add surface modifications to the nanoparticles that facilitate transport across the blood-brain barrier. For example, using sugar-coated polymeric nanoparticles that bind a major glucose transporter in the brain called GLUT-1, researchers successfully targeted coding and noncoding transcripts in the brain following intravenous administration (225, 235). In other studies, exosomes with a transferrin binding ligand attached to the surface effectively delivered antimiRs into the rat brain after an intravenous injection. Systemic delivery of nucleic acid payloads to the brain has also been accomplished using rabies virus glycoprotein (RVG) exosomes and liposomes (236–238), transferrin-targeted cyclodextrins (239), angiopep-2-targeted lipid- and polymer-based nanoparticles (240, 241), and calcium phosphate lipid nanoparticles (242). Thus, as the number of nanoformulations capable of delivering nucleic acids to the brain continues to improve, ncRNA nanocarrier systems warrant further research in SUD models.
Ongoing challenges and outlook
The lipid bilayer is a billion-year-old barrier that prevents large, charged molecules like RNAs from entering the cell. In addition to this barrier, there are other formidable obstacles that protect cells from RNAs including, RNases, the innate immune system, and for neurons, the blood-brain barrier (243). Despite these natural defenses, decades of basic science and clinical research have recently led to multiple FDA-approved nucleic acid-based therapeutics for various indications (244). However, it is clear that we are still in the early days of ncRNA therapeutic development, particularly for SUD, and several issues need to be addressed to move the field forward. First, most preclinical and all clinical experiments exploring ncRNAs in SUD are correlational studies. Additional functional studies that target conserved ncRNAs in sophisticated SUD models will be essential to identify the ncRNA targets with the highest translational potential. Also, as low-quality sequence data have incorrectly annotated some ncRNAs (245, 246), SUD-associated ncRNAs should be thoroughly characterized and validated as true ncRNAs before being pursued therapeutically. To facilitate therapeutic developoment, multiple bioinformatic tools have been created to predict ncRNA targets and assist with characterization and safety (245, 247). Second, rather than studying the ncRNAs involved in the acquisition of drug-seeking, researchers should focus on ncRNA mechanisms that drive drug craving, relapse, and withdrawal, as such targets are likely more relevant to promote abstinence and recovery in humans. Also, as different cells and circuits may exert contrasting effects in the context of SUD, additional cell-type specific studies are needed to identify the most promising ncRNA targets. Third, instead of injecting RNA-based therapeutics directly into the brain in preclinical models, researchers are encouraged to test clinically relevant routes of administration for ncRNA treatments. For example, multiple studies have demonstrated the promise of intranasal administration as a way to bypass the blood-brain barrier (196, 197, 231, 248–253). Intrathecal injections of modified ASOs and siRNAs and nanoparticle-containing nucleic acids have also achieved high brain uptake in preclinical and clinical studies (131, 200, 254) and should also be employed in SUD experiments. Finally, using nucleic acids, nanoparticles, and/or AAVs that contain ligands or surface modifications to promote brain and/or cell type-specific delivery is an approach to enhance CNS uptake and avoid potential side effects (7, 217, 247, 255–257). N-acetylgalactosamine (GalNac), a biomolecule conjugate that promotes liver-specific uptake of RNA-targeted therapeutics, is a prime example of how such modifications can facilitate tissue-specific uptake. Additional research is needed to determine whether similar opportunities exist to enhance CNS-specific delivery.
An additional strategy to move the field forward is to repurpose or test clinical-stage nucleic acid-based therapeutics that may also have relevance to SUD. For example, several companies have developed miR mimics or antimiR that target miRs linked to SUD (28, 53, 64, 73, 74, 82, 83, 258–260). Also, SMIRNA databases (e.g., R-BIND, infoRNA) (261, 262) could be used to identify compounds that target SUD-relevant ncRNAs, an appealing translational approach as small molecules typically have a better physicochemical profile compared to nucleic acids. These databases also consist of clinically tested small molecules, providing drug repurposing opportunities for rapid translational applications. Additionally, the abused substance itself may create opportunities for nucleic acid-based treatments. For example, the disrupted blood-brain barrier caused by chronic methamphetamine use (263) may allow for RNA-based drug delivery via less invasive routes of administration, a hypothesis that merits further exploration.
Although many promising opportunities are listed above, multiple clinical trials using RNA-based treatments have been withdrawn due to severe side effects or limited efficacy (18, 247, 264). These failures may serve as lessons learned for future SUD therapeutics. For instance, in preclinical studies, MRX34, a liposome-delivered miR-34a mimic for treatment of solid tumors, showed favorable efficacy and safety profile (265, 266). However, when injected systemically in humans, MRX34 induced severe immune-related side effects and death in some patients causing the clinical trial to be terminated (264). MRX34 was designed to target the low-pH environment in tumors, but preclinical studies indicated that it also accumulates in the bone marrow and other organs, potentially impacting immune cell activity (267). This incident highlights the need for a thorough risk assessment of all organ systems following systemic administration of RNA therapeutics. In another example, oblimersen, a phosphothiorate-modified ASO targeting BCL2 mRNA, showed promise in preclinical experiments but lacked efficacy in multiple clinical trials (268, 269). Further analyses revealed that several off-target effects of oblimersen were related to the phosphothiorate modification, as these off-target effects were not observed with the same ASO that lacked this modification (270–272). On a related note, the RNA payload may also alter the efficacy of the delivery vehicle. For example, nanoparticle tropism has been shown to change based on the type of cargo (273). Thus, going forward, each RNA modification along with the delivery vehicle should be carefully assessed for efficacy and safety before moving to human subjects.
Dosing is another major issue that needs to be addressed in ncRNA-targeting therapeutics, as many ncRNA studies have used supraphysiological concentrations that may lead to unpredictable side effects (247, 274). For example, high doses of miR mimics can cause off-target effects by saturating RISC, potentially blocking the activity of unrelated miRs and triggering a cascade of side effects. As a prerequisite for clinical studies, future experiments should establish dose-dependent on- and off-target effects of the ncRNA therapeutic in both control and pathological conditions. To address dose-dependent toxicities, metronomic ncRNA therapy is an approach used in cancer in which frequent low doses of the ncRNA therapy are administered (usually in combination with conventional treatments) to avoid excessive toxicity or immunogenicity (275). Similar strategies could also be investigated for efficacy and safety in SUD studies. Finally, the exorbitant price of RNA-based therapeutics is a continuing issue that needs to be addressed, particularly for SUD patients that may lack sufficient means to purchase these costly drugs. Ongoing efforts to address these concerns will open the door for ncRNA SUD therapeutics.
Author contributions
SAS wrote the manuscript with edits and feedback from GCS.
Funding
This publication was supported by National Institute on Drug Abuse grants R00DA040744 and U18DA052394.
Conflict of interest
The authors declare that the research was conducted in the absence of any commercial or financial relationships that could be construed as a potential conflict of interest.
References
1. Degenhardt, L, Charlson, F, Ferrari, A, Santomauro, D, Erskine, H, Mantilla-Herrara, A, et al. The global burden of disease attributable to alcohol and drug use in 195 countries and territories, 1990–2016: A systematic analysis for the global burden of disease study 2016. Lancet Psychiatry (2018) 5(12):987–1012. doi:10.1016/S2215-0366(18)30337-7
2. Butelman, ER, and Kreek, MJ. Medications for substance use disorders (SUD): Emerging approaches. Expert Opin Emerg Drugs (2017) 22:301–15. doi:10.1080/14728214.2017.1395855
3. Clamp, M, Fry, B, Kamal, M, Xie, X, Cuff, J, Lin, MF, et al. Distinguishing protein-coding and noncoding genes in the human genome. Proc Natl Acad Sci U S A (2007) 104(49):19428–33. doi:10.1073/pnas.0709013104
4. Ezkurdia, I, Juan, D, Rodriguez, JM, Frankish, A, Diekhans, M, Harrow, J, et al. Multiple evidence strands suggest that theremay be as few as 19 000 human protein-coding genes. Hum Mol Genet (2014) 23(22):5866–78. doi:10.1093/hmg/ddu309
5. Hopkins, A, and Colin, G. The druggable genome. Nat Rev Drug Discov (2002) 1:727–30. doi:10.1038/nrd892
6. Dammes, N, and Peer, D. Paving the road for RNA therapeutics. Trends Pharmacol Sci (2020) 41:755–75. doi:10.1016/j.tips.2020.08.004
7. Kulkarni, JA, Witzigmann, D, Thomson, SB, Chen, S, Leavitt, BR, Cullis, PR, et al. Author Correction: The current landscape of nucleic acid therapeutics. Nat Nanotechnol (2021) 16:841. doi:10.1038/s41565-021-00937-w
8. Damase, TR, Sukhovershin, R, Boada, C, Taraballi, F, Pettigrew, RI, and Cooke, JP. The limitless future of RNA therapeutics. Front Bioeng Biotechnol (2021) 9:628137. doi:10.3389/fbioe.2021.628137
9. Cech, TR, and Steitz, JA. The noncoding RNA revolution - trashing old rules to forge new ones. Cell (2014) 157:77–94. doi:10.1016/j.cell.2014.03.008
10. Kyzar, EJ, Bohnsack, JP, and Pandey, SC. Current and future perspectives of noncoding RNAs in brain function and neuropsychiatric disease. Biol Psychiatry (2022) 91:183–93. doi:10.1016/j.biopsych.2021.08.013
11. Srinivasan, C, Phan, BN, Lawler, AJ, Ramamurthy, E, Kleyman, M, Brown, AR, et al. Addiction-associated genetic variants implicate brain cell type- and region-specific cis-regulatory elements in addiction neurobiology. J Neurosci (2021) 41(43):9008–30. doi:10.1523/JNEUROSCI.2534-20.2021
12. Ponting, CP, Oliver, PL, and Reik, W. Evolution and functions of long noncoding RNAs. Cell (2009) 136:629–41. doi:10.1016/j.cell.2009.02.006
13. Nie, JH, Li, TX, Zhang, XQ, and Liu, J. Roles of non-coding RNAs in normal human brain development, brain tumor, and neuropsychiatric disorders. Noncoding RNA (2019) 5:E36. doi:10.3390/ncrna5020036
14. Gowen, AM, Odegaard, KE, Hernandez, J, Chand, S, Koul, S, Pendyala, G, et al. Role of microRNAs in the pathophysiology of addiction, 12. Wiley Interdisciplinary Reviews: RNA (2021).
15. Liu, J, Zhou, F, Guan, Y, Meng, F, Zhao, Z, Su, Q, et al. The biogenesis of miRNAs and their role in the development of amyotrophic lateral sclerosis. Cells (2022) 11:572. doi:10.3390/cells11030572
16. Nakamori, M, Junn, E, Mochizuki, H, and Mouradian, MM. Nucleic acid–based therapeutics for Parkinson’s disease. Neurotherapeutics (2019) 16:287–98. doi:10.1007/s13311-019-00714-7
17. Riscado, M, Baptista, B, and Sousa, F. New rna-based breakthroughs in alzheimer’s disease diagnosis and therapeutics. Pharmaceutics (2021) 13:1397. doi:10.3390/pharmaceutics13091397
18. Anastasiadou, E, Seto, AG, Beatty, X, Hermreck, M, Gilles, ME, Stroopinsky, D, et al. Cobomarsen, an oligonucleotide inhibitor of miR-155, slows DLBCL tumor cell growth in vitro and in vivo. Clin Cancer Res (2021) 27(4):1139–49. doi:10.1158/1078-0432.CCR-20-3139
19. Darcq, E, Warnault, V, Phamluong, K, Besserer, GM, Liu, F, and Ron, D. MicroRNA-30a-5p in the prefrontal cortex controls the transition from moderate to excessive alcohol consumption. Mol Psychiatry (2015) 20(10):1219–31. doi:10.1038/mp.2014.120
20. Bahi, A, and Dreyer, JL. Striatal modulation of BDNF expression using microRNA124a-expressing lentiviral vectors impairs ethanol-induced conditioned-place preference and voluntary alcohol consumption. Eur J Neurosci (2013) 38(2):2328–37. doi:10.1111/ejn.12228
21. Wang, Z. The principles of MiRNA-masking antisense oligonucleotides technology. Methods Mol Biol (2011) 676:43–9. doi:10.1007/978-1-60761-863-8_3
22. Li, J, Li, J, Liu, X, Qin, S, Guan, Y, Liu, Y, et al. MicroRNA expression profile and functional analysis reveal that miR-382 is a critical novel gene of alcohol addiction. EMBO Mol Med (2013) 5(9):1402–14. doi:10.1002/emmm.201201900
23. Xu, W, Zhao, M, Lin, Z, Liu, H, Ma, H, Hong, Q, et al. Increased expression of plasma hsa-miR-181a in male patients with heroin addiction use disorder. J Clin Lab Anal (2020) 34(11):e23486. doi:10.1002/jcla.23486
24. Yu, H, Xie, B, Zhang, J, Luo, Y, Galaj, E, Zhang, X, et al. The role of circTmeff-1 in incubation of context-induced morphine craving. Pharmacol Res (2021) 170:105722. doi:10.1016/j.phrs.2021.105722
25. Hollander, JA, Im, HI, Amelio, AL, Kocerha, J, Bali, P, Lu, Q, et al. Striatal microRNA controls cocaine intake through CREB signalling. Nature (2010) 466(7303):197–202. doi:10.1038/nature09202
26. Bastle, RM, Oliver, RJ, Gardiner, AS, Pentkowski, NS, Bolognani, F, Allan, AM, et al. In silico identification and in vivo validation of miR-495 as a novel regulator of motivation for cocaine that targets multiple addiction-related networks in the nucleus accumbens. Mol Psychiatry (2018) 23(2):434–43. doi:10.1038/mp.2016.238
27. Farris, SP, and Dayne Mayfield, R. RNA-seq reveals novel transcriptional reorganization in human alcoholic brain. Int Rev Neurobiol (2014) 116:275–300. doi:10.1016/B978-0-12-801105-8.00011-4
28. Su, H, Zhu, L, Li, J, Wang, R, Liu, D, Han, W, et al. Regulation of microRNA-29c in the nucleus accumbens modulates methamphetamine -induced locomotor sensitization in mice. Neuropharmacology (2019) 148:160–8. doi:10.1016/j.neuropharm.2019.01.007
29. Qian, H, Shang, Q, Liang, M, Gao, B, Xiao, J, Wang, J, et al. MicroRNA-31-3p/RhoA signaling in the dorsal hippocampus modulates methamphetamine-induced conditioned place preference in mice. Psychopharmacology (Berl) (2021) 238:3207–19. doi:10.1007/s00213-021-05936-2
30. Li, J, Zhu, L, Su, H, Liu, D, Yan, Z, Ni, T, et al. Regulation of miR-128 in the nucleus accumbens affects methamphetamine-induced behavioral sensitization by modulating proteins involved in neuroplasticity. Addict Biol (2021) 26(1):e12881. doi:10.1111/adb.12881
31. Mavrikaki, M, Anastasiadou, E, Ozdemir, RA, Potter, D, Helmholz, C, Slack, FJ, et al. Overexpression of miR-9 in the nucleus accumbens increases oxycodone self-administration. Int J Neuropsychopharmacol (2019) 22(6):383–93. doi:10.1093/ijnp/pyz015
32. Jia, M, Wang, X, Zhang, H, Ye, C, Ma, H, Yang, M, et al. MicroRNA-132 in the adult dentate gyrus is involved in opioid addiction via modifying the differentiation of neural stem cells. Neurosci Bull (2019) 35(3):486–96. doi:10.1007/s12264-019-00338-z
33. Irie, T, Shum, R, Deni, I, Hunkele, A, le Rouzic, V, Xu, J, et al. Identification of abundant and evolutionarily conserved opioid receptor circular RNAs in the nervous system modulated by morphine. Mol Pharmacol (2019) 96(2):247–58. doi:10.1124/mol.118.113977
34. Gomez, AM, Altomare, D, Sun, WL, Midde, NM, Ji, H, Shtutman, M, et al. Prefrontal microRNA-221 mediates environmental enrichment-induced increase of locomotor sensitivity to nicotine. Int J Neuropsychopharmacol (2016) 19(1):pyv090. doi:10.1093/ijnp/pyv090
35. Bannon, MJ, Savonen, CL, Jia, H, Dachet, F, Halter, SD, Schmidt, CJ, et al. Identification of long noncoding RNAs dysregulated in the midbrain of human cocaine abusers. J Neurochem (2015) 135(1):50–9. doi:10.1111/jnc.13255
36. Ha, M, and Kim, VN. Regulation of microRNA biogenesis. Nat Rev Mol Cel Biol. (2014) 15:509–24. doi:10.1038/nrm3838
37. Rooij, E, and Kauppinen, S. Development of micro RNA therapeutics is coming of age. EMBO Mol Med (2014) 6(7):851–64. doi:10.15252/emmm.201100899
38. O’Brien, J, Hayder, H, Zayed, Y, and Peng, C. Overview of microRNA biogenesis, mechanisms of actions, and circulation. Front Endocrinol (2018) 9:402. doi:10.3389/fendo.2018.00402
39. Saliminejad, K, Khorram Khorshid, HR, Soleymani Fard, S, and Ghaffari, SH. An overview of microRNAs: Biology, functions, therapeutics, and analysis methods. J Cel Physiol. (2019) 234:5451–65. doi:10.1002/jcp.27486
40. Yapijakis, C. Regulatory role of MicroRNAs in brain development and function. In: Advances in Experimental Medicine and Biology (2020).
41. Gui, YX, Liu, H, Zhang, LS, Lv, W, and Hu, XY. Altered microRNA profiles in cerebrospinal fluid exosome in Parkinson disease and Alzheimer disease. Oncotarget (2015) 6(35):37043–53. doi:10.18632/oncotarget.6158
42. Briggs, CE, Wang, Y, Kong, B, Woo, TUW, Iyer, LK, and Sonntag, KC. Midbrain dopamine neurons in Parkinson’s disease exhibit a dysregulated miRNA and target-gene network. Brain Res (2015) 1618:111–21. doi:10.1016/j.brainres.2015.05.021
43. Im, HI, Hollander, JA, Bali, P, and Kenny, PJ. MeCP2 controls BDNF expression and cocaine intake through homeostatic interactions with microRNA-212. Nat Neurosci (2010) 13(9):1120–7. doi:10.1038/nn.2615
44. Eipper-Mains, JE, Kiraly, DD, Palakodeti, D, Mains, RE, Eipper, BA, and Graveley, BR. microRNA-Seq reveals cocaine-regulated expression of striatal microRNAs. RNA (2011) 17(8):1529–43. doi:10.1261/rna.2775511
45. Chen, CL, Liu, H, and Guan, X. Changes in microRNA expression profile in hippocampus during the acquisition and extinction of cocaine-induced conditioned place preference in rats. J Biomed Sci (2013) 20(1):96. doi:10.1186/1423-0127-20-96
46. Cabana-Domínguez, J, Arenas, C, Cormand, B, and Fernàndez-Castillo, N. MiR-9, miR-153 and miR-124 are down-regulated by acute exposure to cocaine in a dopaminergic cell model and may contribute to cocaine dependence. Transl Psychiatry (2018) 8(1):173. doi:10.1038/s41398-018-0224-5
47. Guo, ML, Periyasamy, P, Liao, K, Kook, YH, Niu, F, Callen, SE, et al. Cocaine-mediated downregulation of microglial miR-124 expression involves promoter DNA methylation. Epigenetics (2016) 11(11):819–30. doi:10.1080/15592294.2016.1232233
48. Sessa, F, Maglietta, F, Bertozzi, G, Salerno, M, di Mizio, G, Messina, G, et al. Human brain injury and mirnas: An experimental study. Int J Mol Sci (2019) 20(7):E1546. doi:10.3390/ijms20071546
49. Sequeira-Cordero, A, and Brenes, JC. Time-dependent changes in striatal monoamine levels and gene expression following single and repeated amphetamine administration in rats. Eur J Pharmacol (2021) 904:174148. doi:10.1016/j.ejphar.2021.174148
50. Cuesta, S, Restrepo-Lozano, JM, Popescu, C, He, S, Reynolds, LM, Israel, S, et al. DCC-related developmental effects of abused- versus therapeutic-like amphetamine doses in adolescence. Addict Biol (2020) 25(4):e12791. doi:10.1111/adb.12791
51. Cuesta, S, Restrepo-Lozano, JM, Silvestrin, S, Nouel, D, Torres-Berrío, A, Reynolds, LM, et al. Non-contingent exposure to amphetamine in adolescence recruits miR-218 to regulate dcc expression in the VTA. Neuropsychopharmacology (2018) 43(4):900–11. doi:10.1038/npp.2017.284
52. Zhu, L, Li, J, Dong, N, Guan, F, Liu, Y, Ma, D, et al. MRNA changes in nucleus accumbens related to methamphetamine addiction in mice. Sci Rep (2016) 6:36993. doi:10.1038/srep36993
53. Bosch, PJ, Benton, MC, Macartney-Coxson, D, and Kivell, BM. mRNA and microRNA analysis reveals modulation of biochemical pathways related to addiction in the ventral tegmental area of methamphetamine self-administering rats. BMC Neurosci (2015) 16(1):43. doi:10.1186/s12868-015-0186-y
54. Du, HY, Cao, DN, Chen, Y, Wang, L, Wu, N, and Li, J. Alterations of prefrontal cortical microRNAs in methamphetamine self-administering rats: From controlled drug intake to escalated drug intake. Neurosci Lett (2016) 611:21–7. doi:10.1016/j.neulet.2015.11.016
55. Sim, MS, Soga, T, Pandy, V, Wu, YS, Parhar, IS, and Mohamed, Z. MicroRNA expression signature of methamphetamine use and addiction in the rat nucleus accumbens. Metab Brain Dis (2017) 32(6):1767–83. doi:10.1007/s11011-017-0061-x
56. Ni, T, Li, Y, Wang, R, Hu, T, Guan, F, Zhu, L, et al. The potential involvement of miR-204-3p-axon guidance network in methamphetamine-induced locomotor sensitization of mice. Neurosci Lett (2019) 707:134303. doi:10.1016/j.neulet.2019.134303
57. Chand, S, Gowen, A, Savine, M, Moore, D, Clark, A, Huynh, W, et al. A comprehensive study to delineate the role of an extracellular vesicle-associated microRNA-29a in chronic methamphetamine use disorder. J Extracell Vesicles (2021) 10(14):e12177. doi:10.1002/jev2.12177
58. Lallai, V, Grimes, N, Fowler, JP, Sequeira, PA, Cartagena, P, Limon, A, et al. Nicotine acts on cholinergic signaling mechanisms to directly modulate choroid plexus function. eNeuro (2019) 6(2):0051–19. doi:10.1523/ENEURO.0051-19.2019
59. Rauthan, M, Gong, J, Liu, J, Li, Z, Wescott, SA, Liu, J, et al. MicroRNA regulation of nAChR expression and nicotine-dependent behavior in C. elegans. Cell Rep. (2017) 21(6):1434–41. doi:10.1016/j.celrep.2017.10.043
60. Keller, RF, Dragomir, A, Yantao, F, Akay, YM, and Akay, M. Investigating the genetic profile of dopaminergic neurons in the VTA in response to perinatal nicotine exposure using mRNA-miRNA analyses. Sci Rep (2018) 8(1):13769. doi:10.1038/s41598-018-31882-9
61. Pittenger, ST, Schaal, VL, Moore, D, Guda, RS, Koul, S, Yelamanchili, Sv., et al. MicroRNA cluster miR199a/214 are differentially expressed in female and male rats following nicotine self-administration. Sci Rep (2018) 8(1):17464. doi:10.1038/s41598-018-35747-z
62. Lippi, G, Steinert, JR, Marczylo, EL, D’Oro, S, Fiore, R, Forsythe, ID, et al. Targeting of the Arpc3 actin nucleation factor by miR-29a/b regulates dendritic spine morphology. J Cel Biol. (2011) 194(6):889–904. doi:10.1083/jcb.201103006
63. Lee, S, Woo, J, Kim, YS, and Im, HI. Integrated miRNA-mRNA analysis in the habenula nuclei of mice intravenously self-administering nicotine. Sci Rep (2015) 5:12909. doi:10.1038/srep12909
64. Kim, J, Im, HI, and Moon, C. Intravenous morphine self-administration alters accumbal microRNA profiles in the mouse brain. Neural Regen Res (2018) 13(1):77–85. doi:10.4103/1673-5374.224374
65. Tapocik, JD, Ceniccola, K, Mayo, CL, Schwandt, ML, Solomon, M, Wang, BD, et al. MicroRNAs are involved in the development of morphine-induced analgesic tolerance and regulate functionally relevant changes in serpini1. Front Mol Neurosci (2016) 9(MAR):20. doi:10.3389/fnmol.2016.00020
66. He, Y, Yang, C, Kirkmire, CM, and Wang, ZJ. Regulation of opioid tolerance by let-7 family microRNA targeting the μ opioid receptor. J Neurosci (2010) 30(30):10251–8. doi:10.1523/JNEUROSCI.2419-10.2010
67. Tapocik, JD, Luu, Tv., Mayo, CL, Wang, BD, Doyle, E, Lee, AD, et al. Neuroplasticity, axonal guidance and micro-RNA genes are associated with morphine self-administration behavior. Addict Biol (2013) 18(3):480–95. doi:10.1111/j.1369-1600.2012.00470.x
68. Xu, W, Hong, Q, Lin, Z, Ma, H, Chen, W, Zhuang, D, et al. Role of nucleus accumbens microRNA-181a and MeCP2 in incubation of heroin craving in male rats. Psychopharmacology (Berl) (2021) 238(8):2313–24. doi:10.1007/s00213-021-05854-3
69. Yan, B, Hu, Z, Yao, W, Le, Q, Xu, B, Liu, X, et al. MiR-218 targets MeCP2 and inhibits heroin seeking behavior. Sci Rep (2017) 7:40413. doi:10.1038/srep40413
70. Wu, Q, Hwang, CK, Zheng, H, Wagley, Y, Lin, HY, Kim, DK, et al. MicroRNA 339 down-regulates μ-opioid receptor at the post-transcriptional level in response to opioid treatment. FASEB J (2013) 27(2):522–35. doi:10.1096/fj.12-213439
71. Shi, X, Li, Y, Yan, P, Shi, Y, and Lai, J. Weighted gene co-expression network analysis to explore the mechanism of heroin addiction in human nucleus accumbens. J Cel Biochem. (2020) 121(2):1870–9. doi:10.1002/jcb.29422
72. Pietrzykowski, AZ, Friesen, RM, Martin, GE, Puig, SI, Nowak, CL, Wynne, PM, et al. Posttranscriptional regulation of BK channel splice variant stability by miR-9 underlies neuroadaptation to alcohol. Neuron (2008) 59(2):274–87. doi:10.1016/j.neuron.2008.05.032
73. Gorini, G, Nunez, YO, and Mayfield, RD. Integration of miRNA and protein profiling reveals coordinated neuroadaptations in the alcohol-dependent mouse brain. PLoS One (2013) 8(12):e82565. doi:10.1371/journal.pone.0082565
74. Asimes, AD, Kim, CK, Rao, YS, Bartelt, K, and Pak, TR. microRNA expression profiles in the ventral hippocampus during pubertal development and the impact of peri-pubertal binge alcohol exposure. Noncoding RNA (2019) 5(1):E21. doi:10.3390/ncrna5010021
75. Tapocik, JD, Barbier, E, Flanigan, M, Solomon, M, Pincus, A, Pilling, A, et al. MicroRNA-206 in rat medial prefrontal cortex regulates BDNF expression and alcohol drinking. J Neurosci (2014) 34(13):4581–8. doi:10.1523/JNEUROSCI.0445-14.2014
76. Santos-Bezerra, DP, Cavaleiro, AM, Santos, AS, Suemoto, CK, Pasqualucci, CA, Jacob-Filho, W, et al. Alcohol use disorder is associated with upregulation of MicroRNA-34a and MicroRNA-34c in hippocampal postmortem tissue. Alcohol Clin Exp Res (2021) 45(1):64–8. doi:10.1111/acer.14505
77. Manzardo, AM, Gunewardena, S, and Butler, MG. Over-expression of the miRNA cluster at chromosome 14q32 in the alcoholic brain correlates with suppression of predicted target mRNA required for oligodendrocyte proliferation. Gene (2013) 526(2):356–63. doi:10.1016/j.gene.2013.05.052
78. Zhang, H, Wang, F, Xu, H, Liu, Y, Liu, J, Zhao, H, et al. Differentially co-expressed genes in postmortem prefrontal cortex of individuals with alcohol use disorders: Influence on alcohol metabolism-related pathways. Hum Genet (2014) 133(11):1383–94. doi:10.1007/s00439-014-1473-x
79. Zhang, JG, Gelernter, J, and Zhang, H. Differential expression of miR-130a in postmortem prefrontal cortex of subjects with alcohol use disorders. J Addict Res Ther (2013) 04(04):18179. doi:10.4172/2155-6105.1000155
80. Vornholt, E, Drake, J, Mamdani, M, McMichael, G, Taylor, ZN, Bacanu, SA, et al. Network preservation reveals shared and unique biological processes associated with chronic alcohol abuse in NAc and PFC. PLoS One (2020) 15(12):e0243857. doi:10.1371/journal.pone.0243857
81. Lim, Y, Beane-Ebel, JE, Tanaka, Y, Ning, B, Husted, CR, Henderson, DC, et al. Exploration of alcohol use disorder-associated brain miRNA–mRNA regulatory networks. Transl Psychiatry (2021) 11(1):504. doi:10.1038/s41398-021-01635-w
82. Lewohl, JM, Nunez, YO, Dodd, PR, Tiwari, GR, Harris, RA, and Mayfield, RD. Up-regulation of microRNAs in brain of human alcoholics. Alcohol Clin Exp Res (2011) 35(11):1928–37. doi:10.1111/j.1530-0277.2011.01544.x
83. Mamdani, M, Williamson, V, McMichael, GO, Blevins, T, Aliev, F, Adkins, A, et al. Integrating mRNA and miRNA weighted gene co-expression networks with eQTLs in the nucleus accumbens of subjects with alcohol dependence. PLoS One (2015) 10(9):e0137671. doi:10.1371/journal.pone.0137671
84. Zhao, Y, Qin, F, Han, S, Li, S, Zhao, Y, Wang, H, et al. MicroRNAs in drug addiction: Current status and future perspectives. Pharmacol Ther (2022) 236:108215. doi:10.1016/j.pharmthera.2022.108215
85. Liu, H, Xu, W, Feng, J, Ma, H, Zhang, J, Xie, X, et al. Corrigendum: Increased expression of plasma miRNA-320a and let-7b-5p in heroin-dependent patients and its clinical significance. Front Psychiatry (2021) 12:733293. doi:10.3389/fpsyt.2021.733293
86. Hsu, CW, Huang, TL, and Tsai, MC. Decreased level of blood microRNA-133b in men with opioid use disorder on methadone maintenance therapy. J Clin Med (2019) 8(8):E1105. doi:10.3390/jcm8081105
87. Toyama, K, Kiyosawa, N, Watanabe, K, and Ishizuka, H. Identification of circulating miRNAs differentially regulated by opioid treatment. Int J Mol Sci (2017) 18(9):E1991. doi:10.3390/ijms18091991
88. Zhao, Y, Zhang, K, Jiang, H, Du, J, Na, Z, Hao, W, et al. Decreased expression of plasma MicroRNA in patients with methamphetamine (MA) use disorder. J Neuroimmune Pharmacol (2016) 11(3):542–8. doi:10.1007/s11481-016-9671-z
89. Gu, WJ, Zhang, C, Zhong, Y, Luo, J, Zhang, CY, Zhang, C, et al. Altered serum microRNA expression profile in subjects with heroin and methamphetamine use disorder. Biomed Pharmacother (2020) 125.
90. Ignacio, C, Hicks, SD, Burke, P, Lewis, L, Szombathyne-Meszaros, Z, and Middleton, FA. Alterations in serum microRNA in humans with alcohol use disorders impact cell proliferation and cell death pathways and predict structural and functional changes in brain. BMC Neurosci (2015) 16(1):55. doi:10.1186/s12868-015-0195-x
91. ten Berg, PW, Shaffer, J, Vliegenthart, ADB, McCrae, J, Sharkey, N, Webb, DJ, et al. Attending a social event and consuming alcohol is associated with changes in serum microRNA: A before and after study in healthy adults. Biomarkers (2018) 23(8):781–6. doi:10.1080/1354750X.2018.1499128
92. Sugiura, T, Dohi, Y, Yamashita, S, Iwaki, S, Ito, S, Sanagawa, A, et al. Circulating level of microRNA-126 may be a potential biomarker for recovery from smoking-related vascular damage in middle-aged habitual smokers. Int J Cardiol Heart Vasc (2015) 7:83–7. doi:10.1016/j.ijcha.2015.02.012
93. Kim, B, Tag, SH, Kim, YS, Cho, SN, and Im, HI. Circulating microRNA miR-137 as a stable biomarker for methamphetamine abstinence. Psychopharmacology (Berl) (2022) 239(3):831–40. doi:10.1007/s00213-022-06074-z
94. Viola, TW, Heberle, BA, Zaparte, A, Sanvicente-Vieira, B, Wainer, LM, Fries, GR, et al. Peripheral blood microRNA levels in females with cocaine use disorder. J Psychiatr Res (2019) 114:48–54. doi:10.1016/j.jpsychires.2019.03.028
95. Chandrasekar, V, and Dreyer, JL. Regulation of MiR-124, Let-7d, and MiR-181a in the accumbens affects the expression, extinction, and reinstatement of cocaine-induced conditioned place preference. Neuropsychopharmacology (2011) 36(6):1149–64. doi:10.1038/npp.2010.250
96. Quinn, RK, Brown, AL, Goldie, BJ, Levi, EM, Dickson, PW, Smith, DW, et al. Distinct miRNA expression in dorsal striatal subregions is associated with risk for addiction in rats. Transl Psychiatry (2015) 5(2):e503. doi:10.1038/tp.2014.144
97. Briggs, JA, Wolvetang, EJ, Mattick, JS, Rinn, JL, and Barry, G. Mechanisms of long non-coding RNAs in mammalian nervous system development, plasticity, disease, and evolution. Plasticity, Dis EvolutionNeuron (2015) 88:861–77. doi:10.1016/j.neuron.2015.09.045
98. Andersen, RE, and Lim, DA. Forging our understanding of lncRNAs in the brain. Cell Tissue Res. (2018) 371:55–71. doi:10.1007/s00441-017-2711-z
99. Begolli, R, Sideris, N, and Giakountis, A. Lncrnas as chromatin regulators in cancer: From molecular function to clinical potential. Cancers (2019) 11:E1524. doi:10.3390/cancers11101524
100. Khalil, AM, Guttman, M, Huarte, M, Garber, M, Raj, A, Morales, DR, et al. Many human large intergenic noncoding RNAs associate with chromatin-modifying complexes and affect gene expression. Proc Natl Acad Sci U S A (2009) 106(28):11667–72. doi:10.1073/pnas.0904715106
101. Michelhaugh, SK, Lipovich, L, Blythe, J, Jia, H, Kapatos, G, and Bannon, MJ. Mining Affymetrix microarray data for long non-coding RNAs: Altered expression in the nucleus accumbens of heroin abusers. J Neurochem (2011) 116(3):459–66. doi:10.1111/j.1471-4159.2010.07126.x
102. Mercer, TR, Qureshi, IA, Gokhan, S, Dinger, ME, Li, G, Mattick, JS, et al. Long noncoding RNAs in neuronal-glial fate specification and oligodendrocyte lineage maturation. BMC Neurosci (2010) 11:14. doi:10.1186/1471-2202-11-14
103. Zhang, X, Hamblin, MH, and Yin, KJ. The long noncoding RNA Malat1: Its physiological and pathophysiological functions. RNA Biol (2017) 14:1705–14. doi:10.1080/15476286.2017.1358347
104. Zhao, J, Zhang, X, Zhou, Y, Ansell, PJ, and Klibanski, A. Cyclic AMP stimulates MEG3 gene expression in cells through a cAMP-response element (CRE) in the MEG3 proximal promoter region. Int J Biochem Cel Biol. (2006) 38(10):1808–20. doi:10.1016/j.biocel.2006.05.004
105. Zhu, L, Zhu, J, Liu, Y, Chen, Y, Li, Y, Huang, L, et al. Methamphetamine induces alterations in the long non-coding RNAs expression profile in the nucleus accumbens of the mouse. BMC Neurosci (2015) 16(1):18. doi:10.1186/s12868-015-0157-3
106. Ribeiro, EA, Scarpa, JR, Garamszegi, SP, Kasarskis, A, Mash, DC, and Nestler, EJ. Gene network dysregulation in dorsolateral prefrontal cortex neurons of humans with cocaine use disorder. Sci Rep (2017) 7(1):5412. doi:10.1038/s41598-017-05720-3
107. Saad, MH, Rumschlag, M, Guerra, MH, Savonen, CL, Jaster, AM, Olson, PD, et al. Differentially expressed gene networks, biomarkers, long noncoding RNAs, and shared responses with cocaine identified in the midbrains of human opioid abusers. Sci Rep (2019) 9(1):1534. doi:10.1038/s41598-018-38209-8
108. Farris, SP, Arasappan, D, Hunicke-Smith, S, Harris, RA, and Mayfield, RD. Transcriptome organization for chronic alcohol abuse in human brain. Mol Psychiatry (2015) 20(11):1438–47. doi:10.1038/mp.2014.159
109. van Booven, D, Li, M, Sunil Rao, J, Blokhin, IO, Dayne Mayfield, R, Barbier, E, et al. Alcohol use disorder causes global changes in splicing in the human brain. Transl Psychiatry (2021) 11(1):2. doi:10.1038/s41398-020-01163-z
110. Drake, J, McMichael, GO, Vornholt, ES, Cresswell, K, Williamson, V, Chatzinakos, C, et al. Assessing the role of long noncoding RNA in nucleus accumbens in subjects with alcohol dependence. Alcohol Clin Exp Res (2020) 44(12):2468–80. doi:10.1111/acer.14479
111. Xu, H, Brown, AN, Waddell, NJ, Liu, X, Kaplan, GJ, Chitaman, JM, et al. Role of long noncoding RNA Gas5 in cocaine action. Biol Psychiatry (2020) 88(10):758–66. doi:10.1016/j.biopsych.2020.05.004
112. Sartor, GC, stLaurent, G, and Wahlestedt, C. The emerging role of non-coding RNAs in drug addiction. Front Genet (2012) 3:106. doi:10.3389/fgene.2012.00106
113. Sartor, GC, Powell, SK, Velmeshev, D, Lin, DY, Magistri, M, Wiedner, HJ, et al. Cocaine alters Homer1 natural antisense transcript in the nucleus accumbens. Mol Cel Neurosci. (2017) 85:183–9. doi:10.1016/j.mcn.2017.10.003
114. Bohnsack, JP, Teppen, T, Kyzar, EJ, Dzitoyeva, S, and Pandey, SC. The lncRNA BDNF-AS is an epigenetic regulator in the human amygdala in early onset alcohol use disorders. Transl Psychiatry (2019) 9(1):34. doi:10.1038/s41398-019-0367-z
115. Youngson, NA, Castino, MR, Stuart, A, Kershaw, KA, Holmes, NM, Heffernan, EC, et al. A role for a novel natural antisense-BDNF in the maintenance of nicotine-seeking. Addict Neurosci (2022) 2:100010. doi:10.1016/j.addicn.2022.100010
116. Zheng, X, Lin, C, Li, Y, Ye, J, Zhou, J, and Guo, P. Long noncoding RNA BDNF-AS regulates ketamine-induced neurotoxicity in neural stem cell derived neurons. Biomed Pharmacother (2016) 82:722–8. doi:10.1016/j.biopha.2016.05.050
117. Mahmoudi, E, and Cairns, MJ. Circular RNAs are temporospatially regulated throughout development and ageing in the rat. Sci Rep (2019) 9(1):2564. doi:10.1038/s41598-019-38860-9
118. You, X, Vlatkovic, I, Babic, A, Will, T, Epstein, I, Tushev, G, et al. Neural circular RNAs are derived from synaptic genes and regulated by development and plasticity. Nat Neurosci (2015) 18(4):603–10. doi:10.1038/nn.3975
119. Chen, X, Zhou, M, Yant, L, and Huang, C. Circular RNA in disease: Basic properties and biomedical relevance. Wiley Interdiscip Rev RNA (2022) 22:e1723. n/a(n/a). doi:10.1002/wrna.1723
120. Holdt, LM, Kohlmaier, A, and Teupser, D. Circular RNAs as therapeutic agents and targets. Front Physiol (2018) 9:1262. doi:10.3389/fphys.2018.01262
121. Vornholt, E, Drake, J, Mamdani, M, McMichael, G, Taylor, ZN, Bacanu, SA, et al. Identifying a novel biological mechanism for alcohol addiction associated with circRNA networks acting as potential miRNA sponges. Addict Biol (2021) 26:e13071. doi:10.1111/adb.13071
122. Paudel, P, Pierotti, C, Lozano, E, Amoah, SK, Gardiner, AS, Caldwell, KK, et al. Prenatal alcohol exposure results in sex-specific alterations in circular RNA expression in the developing mouse brain. Front Neurosci (2020) 14:581895. doi:10.3389/fnins.2020.581895
123. Floris, G, Gillespie, A, Zanda, MT, Dabrowski, KR, and Sillivan, SE. Heroin regulates orbitofrontal circular RNAs. Int J Mol Sci (2022) 23(3):1453. doi:10.3390/ijms23031453
124. Shen, Q, Xie, B, Galaj, E, Yu, H, Li, X, Lu, Y, et al. CircTmeff-1 in the nucleus accumbens regulates the reconsolidation of cocaine-associated memory. Brain Res Bull (2022) 185:64–73. Internet. doi:10.1016/j.brainresbull.2022.04.010
125. Bu, Q, Long, H, Shao, X, Gu, H, Kong, J, Luo, L, et al. Cocaine induces differential circular RNA expression in striatum. Transl Psychiatry (2019) 9(1):199. doi:10.1038/s41398-019-0527-1
126. Chen, Y, Li, X, Meng, S, Huang, S, Chang, S, and Shi, J. Identification of functional CircRNA-miRNA-mRNA regulatory network in dorsolateral prefrontal cortex neurons of patients with cocaine use disorder. Front Mol Neurosci (2022) 15. doi:10.3389/fnmol.2022.839233
127. Li, J, Shi, Q, Wang, Q, Tan, X, Pang, K, Liu, X, et al. Profiling circular RNA in methamphetamine-treated primary cortical neurons identified novel circRNAs related to methamphetamine addiction. Neurosci Lett (2019) 701:146–53. doi:10.1016/j.neulet.2019.02.032
128. Li, J, Sun, Q, Zhu, S, Xi, K, Shi, Q, Pang, K, et al. Knockdown of circHomer1 ameliorates METH-induced neuronal injury through inhibiting Bbc3 expression. Neurosci Lett (2020) 732:135050. doi:10.1016/j.neulet.2020.135050
129. Zogg, H, Singh, R, and Ro, S. Current advances in RNA therapeutics for human diseases. Int J Mol Sci (2022) 23:2736. doi:10.3390/ijms23052736
130. Disney, MD. Targeting RNA with small molecules to capture opportunities at the intersection of chemistry, biology, and medicine. J Am Chem Soc (2019) 141:6776–90. doi:10.1021/jacs.8b13419
131. Khorkova, O, Hsiao, J, and Wahlestedt, C. Nucleic acid–based therapeutics in orphan neurological disorders: Recent developments. Front Mol Biosci (2021) 8:643681. doi:10.3389/fmolb.2021.643681
132. Relizani, K, and Goyenvalle, A. Use of tricyclo-DNA antisense oligonucleotides for exon skipping. Methods Mol Biol (2018) 1828:381–94. doi:10.1007/978-1-4939-8651-4_24
133. Crooke, ST, and Bennett, CF. Progress in antisense oligonucleotide therapeutics. Annu Rev Pharmacol Toxicol (1996) 36:107–29. doi:10.1146/annurev.pa.36.040196.000543
134. Ochoa, S, and Milam, VT. Modified nucleic acids: Expanding the capabilities of functional oligonucleotides. Molecules (2020) 25:E4659. doi:10.3390/molecules25204659
135. Hersh, DS, Wadajkar, AS, Roberts, N, Perez, JG, Connolly, NP, Frenkel, V, et al. Evolving drug delivery strategies to overcome the blood brain barrier. Curr Pharm Des (2016) 22(9):1177–93. doi:10.2174/1381612822666151221150733
136. Meng, L, Ward, AJ, Chun, S, Bennett, CF, Beaudet, AL, and Rigo, F. Towards a therapy for Angelman syndrome by targeting a long non-coding RNA. Nature (2015) 518(7539):409–12. doi:10.1038/nature13975
137. Barry, G, Briggs, JA, Vanichkina, DP, Poth, EM, Beveridge, NJ, Ratnu, VS, et al. The long non-coding RNA Gomafu is acutely regulated in response to neuronal activation and involved in schizophrenia-associated alternative splicing. Mol Psychiatry (2014) 19(4):486–94. doi:10.1038/mp.2013.45
138. Zhang, X, Tang, X, Liu, K, Hamblin, MH, and Yin, KJ. Long noncoding RNA malat1 regulates cerebrovascular pathologies in ischemic stroke. J Neurosci (2017) 37(7):1797–806. doi:10.1523/JNEUROSCI.3389-16.2017
139. Michalik, KM, You, X, Manavski, Y, Doddaballapur, A, Zörnig, M, Braun, T, et al. Long noncoding RNA MALAT1 regulates endothelial cell function and vessel growth. Circ Res (2014) 114(9):1389–97. doi:10.1161/CIRCRESAHA.114.303265
140. Amodio, N, Stamato, MA, Juli, G, Morelli, E, Fulciniti, M, Manzoni, M, et al. Drugging the lncRNA MALAT1 via LNA gapmeR ASO inhibits gene expression of proteasome subunits and triggers anti-multiple myeloma activity. Leukemia (2018) 32(9):1948–57. doi:10.1038/s41375-018-0067-3
141. Modarresi, F, Faghihi, MA, Lopez-Toledano, MA, Fatemi, RP, Magistri, M, Brothers, SP, et al. Inhibition of natural antisense transcripts in vivo results in gene-specific transcriptional upregulation. Nat Biotechnol (2012) 30(5):453–9. doi:10.1038/nbt.2158
142. Shum, K, and Rossi, J. In: Methods in Molecular Biology, 1364 (2016).SiRNA Delivery Methods: Methods and Protocols
143. Snead, NM, Escamilla-Powers, JR, Rossi, JJ, and McCaffrey, AP. 5′ unlocked nucleic acid modification improves siRNA targeting. Mol Ther Nucleic Acids (2013) 2:e103. doi:10.1038/mtna.2013.36
144. Robbins, M, Judge, A, Liang, L, McClintock, K, Yaworski, E, and MacLachlan, I. 2′-O-methyl-modified RNAs act as TLR7 antagonists. Mol Ther (2007) 15(9):1663–9. doi:10.1038/sj.mt.6300240
145. Lennox, KA, and Behlke, MA. Cellular localization of long non-coding RNAs affects silencing by RNAi more than by antisense oligonucleotides. Nucleic Acids Res (2016) 44(2):863–77. doi:10.1093/nar/gkv1206
146. Hamil, AS, and Dowdy, SF. Synthesis and conjugation of small interfering ribonucleic neutral siRNNs. Methods Mol Biol (2016) 1364:1–9. doi:10.1007/978-1-4939-3112-5_1
147. Sheridan, C. Billion-dollar deal propels RNAi to CNS frontier. Nat Biotechnol (2019) 37:702–4. doi:10.1038/d41587-019-00014-7
148. Brown, KM, Nair, JK, Janas, MM, Anglero-Rodriguez, YI, Dang, LTH, Peng, H, et al. Expanding RNAi therapeutics to extrahepatic tissues with lipophilic conjugates. Nat Biotechnol (2022) 40:1500–8. Internet. doi:10.1038/s41587-022-01334-x
149. Deng, M, Zhang, Z, Xing, M, Liang, X, Li, Z, Wu, J, et al. LncRNA MRAK159688 facilitates morphine tolerance by promoting REST-mediated inhibition of mu opioid receptor in rats. Neuropharmacology (2022) 206:108938. doi:10.1016/j.neuropharm.2021.108938
150. Huang, R, Zhang, Y, Han, B, Bai, Y, Zhou, R, Gan, G, et al. Circular RNA HIPK2 regulates astrocyte activation via cooperation of autophagy and ER stress by targeting MIR124–2HG. Autophagy (2017) 13(10):1722–41. doi:10.1080/15548627.2017.1356975
151. Liu, S, Cui, B, Dai, Z-X, Shi, P-k, Wang, Z-H, and Guo, Y-Y. Long non-coding RNA HOTAIR promotes Parkinson’;s disease induced by MPTP through up-regulating the expression of LRRK2. Curr Neurovasc Res (2016) 13(2):115–20. doi:10.2174/1567202613666160316155228
152. Cai, L, Tu, L, Li, T, Yang, X, Ren, Y, Gu, R, et al. Downregulation of lncRNA UCA1 ameliorates the damage of dopaminergic neurons, reduces oxidative stress and inflammation in Parkinson’s disease through the inhibition of the PI3K/Akt signaling pathway. Int Immunopharmacol (2019) 75:105734. doi:10.1016/j.intimp.2019.105734
153. Lopez-Toledano, MA, Modarresi, F, Faghihi, MA, Patel, NS, Sahagan, BG, and Wahlestedt, C. Knockdown of BACE1-AS nonprotein-coding transcript modulates beta-amyloid-related hippocampal neurogenesis. Int J Alzheimers Dis (2011) 2011:929042. doi:10.4061/2011/929042
154. Zhang, W, Zhao, H, Wu, Q, Xu, W, and Xia, M. Knockdown of BACE1-AS by siRNA improves memory and learning behaviors in Alzheimer’s disease animal model. Exp Ther Med (2018) 16(3):2080–6. doi:10.3892/etm.2018.6359
155. Li, F, Wang, Y, Yang, H, Xu, Y, Zhou, X, Zhang, X, et al. The effect of BACE1-AS on β-amyloid generation by regulating BACE1 mRNA expression. BMC Mol Biol (2019) 20(1):23. doi:10.1186/s12867-019-0140-0
156. Shao, L, Jiang, G-T, Yang, X-L, Zeng, M-L, Cheng, J-J, Kong, S, et al. Silencing of circIgf1r plays a protective role in neuronal injury via regulating astrocyte polarization during epilepsy. FASEB J (2021) 35(2):e21330. doi:10.1096/fj.202001737RR
157. Wu, Q, and Yi, X. Down-regulation of long noncoding RNA MALAT1 protects hippocampal neurons against excessive autophagy and apoptosis via the PI3K/akt signaling pathway in rats with epilepsy. J Mol Neurosci (2018) 65(2):234–45. doi:10.1007/s12031-018-1093-3
158. Zhao, JH, Wang, B, Wang, XH, Wang, JR, and Xu, CW. Influence of lncRNA ANRIL on neuronal apoptosis in rats with cerebral infarction by regulating the NF-κB signaling pathway. Eur Rev Med Pharmacol Sci (2019) 23(22):10092–100. doi:10.26355/eurrev_201911_19577
159. Zhao, JH, Wang, B, Wang, XH, and Xu, CW. Effect of lncRNA GAS5 on the apoptosis of neurons via the notch1 signaling pathway in rats with cerebral infarction. Eur Rev Med Pharmacol Sci (2019) 23(22):10083–91. doi:10.26355/eurrev_201911_19576
160. Rupaimoole, R, and Slack, FJ. MicroRNA therapeutics: Towards a new era for the management of cancer and other diseases. Nat Rev Drug Discov (2017) 16:203–22. doi:10.1038/nrd.2016.246
161. Jung, J, Yeom, C, Choi, YS, Kim, S, Lee, EJ, Park, MJ, et al. Simultaneous inhibition of multiple oncogenic miRNAs by a multi-potent microRNA sponge. Oncotarget (2015) 6(24):20370–87. doi:10.18632/oncotarget.4827
162. Das, S, Kohr, M, Dunkerly-Eyring, B, Lee, DI, Bedja, D, Kent, OA, et al. Divergent effects of miR-181 family members on myocardial function through protective cytosolic and detrimental mitochondrial microRNA targets. J Am Heart Assoc (2017) 6(3):e004694. doi:10.1161/JAHA.116.004694
163. Bernardo, BC, Gregorevic, P, Ritchie, RH, and McMullen, JR. Generation of MicroRNA-34 sponges and tough decoys for the heart: Developments and challenges. Front Pharmacol (2018) 9:1090. doi:10.3389/fphar.2018.01090
164. Kyzar, EJ, Bohnsack, JP, Zhang, H, and Pandey, SC. MicroRNA-137 drives epigenetic reprogramming in the adult amygdala and behavioral changes after adolescent alcohol exposure. eNeuro (2019) 6(6):0401–19. doi:10.1523/ENEURO.0401-19.2019
165. Most, D, Salem, NA, Tiwari, GR, Blednov, YA, Mayfield, RD, and Harris, RA. Silencing synaptic MicroRNA-411 reduces voluntary alcohol consumption in mice. Addict Biol (2019) 24(4):604–16. doi:10.1111/adb.12625
166. Teppen, TL, Krishnan, HR, Zhang, H, Sakharkar, AJ, and Pandey, SC. The potential role of amygdaloid MicroRNA-494 in alcohol-induced anxiolysis. Biol Psychiatry (2016) 80(9):711–9. doi:10.1016/j.biopsych.2015.10.028
167. Huang, J, Liang, X, Wang, J, Kong, Y, Zhang, Z, Ding, Z, et al. miR-873a-5p targets A20 to facilitate morphine tolerance in mice. Front Neurosci (2019) 13(APR):347. doi:10.3389/fnins.2019.00347
168. Perumal, N, Kanchan, RK, Doss, D, Bastola, N, Atri, P, Chirravuri-Venkata, R, et al. MiR-212-3p functions as a tumor suppressor gene in group 3 medulloblastoma via targeting nuclear factor I/B (NFIB). Acta Neuropathol Commun (2021) 9(1):195. doi:10.1186/s40478-021-01299-z
169. Vázquez-Ríos, AJ, Molina-Crespo, Á, Bouzo, BL, López-López, R, Moreno-Bueno, G, and de la Fuente, M. Exosome-mimetic nanoplatforms for targeted cancer drug delivery. J Nanobiotechnology (2019) 17(1):85. doi:10.1186/s12951-019-0517-8
170. Ofek, P, Calderón, M, Mehrabadi, FS, Krivitsky, A, Ferber, S, Tiram, G, et al. Restoring the oncosuppressor activity of microRNA-34a in glioblastoma using a polyglycerol-based polyplex. Nanomedicine (2016) 12(7):2201–14. doi:10.1016/j.nano.2016.05.016
171. Janssen, HLA, Reesink, HW, Lawitz, EJ, Zeuzem, S, Rodriguez-Torres, M, Patel, K, et al. Treatment of HCV infection by targeting MicroRNA. N Engl J Med (2013) 368(18):1685–94. doi:10.1056/NEJMoa1209026
172. van der Ree, MH, de Vree, JM, Stelma, F, Willemse, S, van der Valk, M, Rietdijk, S, et al. Safety, tolerability, and antiviral effect of RG-101 in patients with chronic hepatitis C: A phase 1B, double-blind, randomised controlled trial. Lancet (2017) 389(10070):709–17. doi:10.1016/S0140-6736(16)31715-9
173. Reid, G, Pel, ME, Kirschner, MB, Cheng, YY, Mugridge, N, Weiss, J, et al. Restoring expression of miR-16: A novel approach to therapy for malignant pleural mesothelioma. Ann Oncol (2013) 24(12):3128–35. doi:10.1093/annonc/mdt412
174. Chakraborty, C, Sharma, AR, Sharma, G, and Lee, SS. Therapeutic advances of miRNAs: A preclinical and clinical update. J Adv Res (2021) 28:127–38. doi:10.1016/j.jare.2020.08.012
175. Romano, G, Acunzo, M, and Nana-Sinkam, P. Micrornas as novel therapeutics in cancer. Cancers (2021) 13:1526. doi:10.3390/cancers13071526
176. Falese, JP, Donlic, A, and Hargrove, AE. Targeting RNA with small molecules: From fundamental principles towards the clinic. Chem Soc Rev (2021) 50:2224–43. doi:10.1039/d0cs01261k
177. Costales, MG, Haga, CL, Velagapudi, SP, Childs-Disney, JL, Phinney, DG, and Disney, MD. Small molecule inhibition of microRNA-210 reprograms an oncogenic hypoxic circuit. J Am Chem Soc (2017) 139(9):3446–55. doi:10.1021/jacs.6b11273
178. Costales, MG, Aikawa, H, Li, Y, Childs-Disney, JL, Abegg, D, Hoch, DG, et al. Small-molecule targeted recruitment of a nuclease to cleave an oncogenic RNA in a mouse model of metastatic cancer. Proc Natl Acad Sci U S A (2020) 117(5):2406–11. doi:10.1073/pnas.1914286117
179. Wang, L, Rowe, RG, Jaimes, A, Yu, C, Nam, Y, Pearson, DS, et al. Small-molecule inhibitors disrupt let-7 oligouridylation and release the selective blockade of let-7 processing by LIN28. Cel Rep. (2018) 23(10):3091–101. doi:10.1016/j.celrep.2018.04.116
180. Chhabra, M. Biological therapeutic modalities. In: Translational Biotechnology: A Journey from Laboratory to Clinics (2021).
181. Warner, KD, Hajdin, CE, and Weeks, KM. Principles for targeting RNA with drug-like small molecules. Nat Rev Drug Discov (2018) 17(8):547–58. doi:10.1038/nrd.2018.93
182. Sztuba-Solinska, J, Chavez-Calvillo, G, and Cline, SE. Unveiling the druggable RNA targets and small molecule therapeutics. Bioorg Med Chem (2019) 27:2149–65. doi:10.1016/j.bmc.2019.03.057
183. Luo, Y, and Disney, MD. Bottom-up design of small molecules that stimulate exon 10 skipping in mutant MAPT pre-mRNA. ChemBioChem (2015) 16(17):2041–4. doi:10.1002/cbic.201402069
184. Keller, CG, Shin, Y, Monteys, AM, Renaud, N, Beibel, M, Teider, N, et al. An orally available, brain penetrant, small molecule lowers huntingtin levels by enhancing pseudoexon inclusion. Nat Commun (2022) 13(1):1150. doi:10.1038/s41467-022-28653-6
185. Disney, MD, Liu, B, Yang, WY, Sellier, C, Tran, T, Charlet-Berguerand, N, et al. A small molecule that targets r(CGG)exp and improves defects in fragile X-associated tremor ataxia syndrome. ACS Chem Biol (2012) 7(10):1711–8. doi:10.1021/cb300135h
186. Yang, WY, He, F, Strack, RL, Oh, SY, Frazer, M, Jaffrey, SR, et al. Small molecule recognition and tools to study modulation of r(CGG)exp in fragile X-associated tremor ataxia syndrome. ACS Chem Biol (2016) 11(9):2456–65. doi:10.1021/acschembio.6b00147
187. Yang, WY, Gao, R, Southern, M, Sarkar, PS, and Disney, MD. Design of a bioactive small molecule that targets r(AUUCU) repeats in spinocerebellar ataxia 10. Nat Commun (2016) 7:11647. doi:10.1038/ncomms11647
188. Rzuczek, SG, Colgan, LA, Nakai, Y, Cameron, MD, Furling, D, Yasuda, R, et al. Precise small-molecule recognition of a toxic CUG RNA repeat expansion. Nat Chem Biol (2017) 13(2):188–93. doi:10.1038/nchembio.2251
189. di Giorgio, A, and Duca, M. Synthetic small-molecule RNA ligands: Future prospects as therapeutic agents. MedChemComm (2019) 10(8):1242–55. doi:10.1039/c9md00195f
190. Dhillon, S. Risdiplam: First approval. Drugs (2020) 80(17):1853–8. doi:10.1007/s40265-020-01410-z
191. Donlic, A, Zafferani, M, Padroni, G, Puri, M, and Hargrove, AE. Regulation of MALAT1 triple helix stability and in vitro degradation by diphenylfurans. Nucleic Acids Res (2020) 48(14):7653–64. doi:10.1093/nar/gkaa585
192. Donlic, A, Morgan, BS, Xu, JL, Liu, A, Roble, C, and Hargrove, AE. Discovery of small molecule ligands for MALAT1 by tuning an RNA-binding scaffold. Angew Chem Int Ed Engl (2018) 57(40):13242–7. doi:10.1002/anie.201808823
193. Ahmadi, S, Zobeiri, M, Mohammadi Talvar, S, Masoudi, K, Khanizad, A, Fotouhi, S, et al. Differential expression of H19, BC1, MIAT1, and MALAT1 long non-coding RNAs within key brain reward regions after repeated morphine treatment. Behav Brain Res (2021) 414:113478. doi:10.1016/j.bbr.2021.113478
194. Tazi, J, Begon-Pescia, C, Campos, N, Apolit, C, Garcel, A, and Scherrer, D. Specific and selective induction of miR-124 in immune cells by the quinoline ABX464: A transformative therapy for inflammatory diseases. Drug Discov Today (2021) 26:1030–9. doi:10.1016/j.drudis.2020.12.019
195. Shi, Y, Parag, S, Patel, R, Lui, A, Murr, M, Cai, J, et al. Stabilization of lncRNA GAS5 by a small molecule and its implications in diabetic adipocytes. Cell Chem. Biol. (2019) 26(3):319–30. doi:10.1016/j.chembiol.2018.11.012
196. Padmakumar, S, Jones, G, Khorkova, O, Hsiao, J, Kim, J, Bleier, BS, et al. Osmotic core-shell polymeric implant for sustained BDNF AntagoNAT delivery in CNS using minimally invasive nasal depot (MIND) approach. Biomaterials (2021) 276:120989. doi:10.1016/j.biomaterials.2021.120989
197. Padmakumar, S, Jones, G, Pawar, G, Khorkova, O, Hsiao, J, Kim, J, et al. Minimally invasive nasal depot (MIND) technique for direct BDNF AntagoNAT delivery to the brain. J Controlled Release (2021) 331:176–86. doi:10.1016/j.jconrel.2021.01.027
198. Deverman, BE, Pravdo, PL, Simpson, BP, Kumar, SR, Chan, KY, Banerjee, A, et al. Cre-dependent selection yields AAV variants for widespread gene transfer to the adult brain. Nat Biotechnol (2016) 34(2):204–9. doi:10.1038/nbt.3440
199. Choudhury, SR, Harris, AF, Cabral, DJ, Keeler, AM, Sapp, E, Ferreira, JS, et al. Widespread central nervous system gene transfer and silencing after systemic delivery of novel AAV-AS vector. Mol Ther (2016) 24(4):726–35. doi:10.1038/mt.2015.231
200. Talbot, K, and Wood, MJA. Wrangling RNA: Antisense oligonucleotides for neurological disorders. Sci Transl Med (2019) 11(511):eaay2069. doi:10.1126/scitranslmed.aay2069
201. Nectow, AR, and Nestler, EJ. Viral tools for neuroscience. Nat Rev Neurosci (2020) 21:669–81. doi:10.1038/s41583-020-00382-z
202. Imbert, M, Dias-Florencio, G, and Goyenvalle, A. Viral vector-mediated antisense therapy for genetic diseases. Genes (2017) 8:E51. doi:10.3390/genes8020051
203. Fu, Y, Chen, J, and Huang, Z. Recent progress in microrna-based delivery systems for the treatment of human disease. ExRNA (2019) 1:24. doi:10.1186/s41544-019-0024-y
204. Lundstrom, K. Viral vectors applied for RNAi-based antiviral therapy. Viruses (2020) 12:924. doi:10.3390/v12090924
205. Komatsu, H. Innovative therapeutic approaches for Huntington’s disease: From nucleic acids to GPCR-targeting small molecules. Front Cel Neurosci. (2021) 15:785703. doi:10.3389/fncel.2021.785703
206. Koshimizu, Y, Isa, K, Kobayashi, K, and Isa, T. Double viral vector technology for selective manipulation of neural pathways with higher level of efficiency and safety. Gene Ther (2021) 28(6):339–50. doi:10.1038/s41434-020-00212-y
207. Jüttner, J, Szabo, A, Gross-Scherf, B, Morikawa, RK, Rompani, SB, Hantz, P, et al. Targeting neuronal and glial cell types with synthetic promoter AAVs in mice, non-human primates and humans. Nat Neurosci (2019) 22(8):1345–56. doi:10.1038/s41593-019-0431-2
208. Bedbrook, CN, Deverman, BE, and Gradinaru, V. Viral strategies for targeting the central and peripheral nervous systems. Annu Rev Neurosci (2018) 41:323–48. doi:10.1146/annurev-neuro-080317-062048
209. Borel, F, Gernoux, G, Sun, H, Stock, R, Blackwood, M, Brown, RH, et al. Safe and effective superoxide dismutase 1 silencing using artificial microRNA in macaques. Sci Transl Med (2018) 10(465):eaau6414. doi:10.1126/scitranslmed.aau6414
210. Mueller, C, Berry, JD, McKenna-Yasek, DM, Gernoux, G, Owegi, MA, Pothier, LM, et al. SOD1 suppression with adeno-associated virus and MicroRNA in familial ALS. N Engl J Med (2020) 383(2):151–8. doi:10.1056/NEJMoa2005056
211. Yang, B, Li, S, Wang, H, Guo, Y, Gessler, DJ, Cao, C, et al. Global CNS transduction of adult mice by intravenously delivered rAAVrh.8 and rAAVrh.10 and nonhuman primates by rAAVrh.10. Mol Ther (2014) 2:1299–309. doi:10.1038/mt.2014.68
212. Tung, YT, Peng, KC, Chen, YC, Yen, YP, Chang, M, Thams, S, et al. Mir-17∼92 confers motor neuron subtype differential resistance to ALS-associated degeneration. Cell Stem Cell (2019) 25(2):193–209. doi:10.1016/j.stem.2019.04.016
213. Dirren, E, Aebischer, J, Rochat, C, Towne, C, Schneider, BL, and Aebischer, P. SOD1 silencing in motoneurons or glia rescues neuromuscular function in ALS mice. Ann Clin Transl Neurol (2015) 2(2):167–84. doi:10.1002/acn3.162
214. Martier, R, Sogorb-Gonzalez, M, Stricker-Shaver, J, Hübener-Schmid, J, Keskin, S, Klima, J, et al. Development of an AAV-based MicroRNA gene therapy to treat machado-joseph disease. Mol Ther Methods Clin Dev (2019) 15:343–58. doi:10.1016/j.omtm.2019.10.008
215. Borel, F, Gernoux, G, Cardozo, B, Metterville, JP, Toro Cabreja, GC, Song, L, et al. Therapeutic rAAVrh10 mediated SOD1 silencing in adult SOD1G93A mice and nonhuman primates. Hum Gene Ther (2016) 27(1):19–31. doi:10.1089/hum.2015.122
216. Hudry, E, and Vandenberghe, LH. Therapeutic AAV gene transfer to the nervous system. A Clin RealityNeuron (2019) 101:839–62. doi:10.1016/j.neuron.2019.02.017:
217. Mendonça, MCP, Kont, A, Aburto, MR, Cryan, JF, and O’Driscoll, CM. Advances in the design of (Nano)Formulations for delivery of antisense oligonucleotides and small interfering RNA: Focus on the central nervous system. Mol Pharmaceutics (2021) 18(4):1491–506. doi:10.1021/acs.molpharmaceut.0c01238
218. Ross, KA, Brenza, TM, Binnebose, AM, Phanse, Y, Kanthasamy, AG, Gendelman, HE, et al. Nano-enabled delivery of diverse payloads across complex biological barriers. J Controlled Release (2015) 219:548–59. doi:10.1016/j.jconrel.2015.08.039
219. Yang, J, Luo, S, Zhang, J, Yu, T, Fu, Z, Zheng, Y, et al. Exosome-mediated delivery of antisense oligonucleotides targeting α-synuclein ameliorates the pathology in a mouse model of Parkinson’s disease. Neurobiol Dis (2021) 148:105218. doi:10.1016/j.nbd.2020.105218
220. Mendonça, MCP, Cronin, MF, Cryan, JF, and O’Driscoll, CM. Modified cyclodextrin-based nanoparticles mediated delivery of siRNA for huntingtin gene silencing across an in vitro BBB model. Eur J Pharmaceutics Biopharmaceutics (2021) 169:309–18. doi:10.1016/j.ejpb.2021.11.003
221. Wei, T, Cheng, Q, Min, YL, Olson, EN, and Siegwart, DJ. Systemic nanoparticle delivery of CRISPR-Cas9 ribonucleoproteins for effective tissue specific genome editing. Nat Commun (2020) 11(1):3232. doi:10.1038/s41467-020-17029-3
222. Rungta, RL, Choi, HB, Lin, PJC, Ko, RWY, Ashby, D, Nair, J, et al. Lipid nanoparticle delivery of sirna to silence neuronal gene expression in the brain. Mol Ther Nucleic Acids (2013) 2:e136. doi:10.1038/mtna.2013.65
223. Yoshida, S, Duong, C, Oestergaard, M, Fazio, M, Chen, C, Peralta, R, et al. MXD3 antisense oligonucleotide with superparamagnetic iron oxide nanoparticles: A new targeted approach for neuroblastoma. Nanomedicine (2020) 24:102127. doi:10.1016/j.nano.2019.102127
224. Wang, P, Zheng, X, Guo, Q, Yang, P, Pang, X, Qian, K, et al. Systemic delivery of BACE1 siRNA through neuron-targeted nanocomplexes for treatment of Alzheimer’s disease. J Controlled Release (2018) 279:220–33. doi:10.1016/j.jconrel.2018.04.034
225. Min, HS, Kim, HJ, Naito, M, Ogura, S, Toh, K, Hayashi, K, et al. Systemic brain delivery of antisense oligonucleotides across the blood–brain barrier with a glucose-coated polymeric nanocarrier. Angew Chem Int Ed Engl (2020) 59(21):8173–80. doi:10.1002/anie.201914751
226. Shilo, M, Motiei, M, Hana, P, and Popovtzer, R. Transport of nanoparticles through the blood-brain barrier for imaging and therapeutic applications. Nanoscale (2014) 6(4):2146–52. doi:10.1039/c3nr04878k
227. Sela, H, Cohen, H, Elia, P, Zach, R, Karpas, Z, and Zeiri, Y. Spontaneous penetration of gold nanoparticles through the blood brain barrier (BBB). J Nanobiotechnology (2015) 13(1):71. doi:10.1186/s12951-015-0133-1
228. Fatima, N, Gromnicova, R, Loughlin, J, Sharrack, B, and Male, D. Gold nanocarriers for transport of oligonucleotides across brain endothelial cells. PLoS One (2020) 15(9):e0236611. doi:10.1371/journal.pone.0236611
229. Male, D, and Gromnicova, R. Nanocarriers for delivery of oligonucleotides to the CNS. Int J Mol Sci (2022) 23:760. doi:10.3390/ijms23020760
230. Anselmo, AC, and Mitragotri, S. Nanoparticles in the clinic: An update post COVID-19 vaccines. Bioeng Translational Med (2021) 6(3):e10246. doi:10.1002/btm2.10246
231. Sukumar, UK, Bose, RJC, Malhotra, M, Babikir, HA, Afjei, R, Robinson, E, et al. Intranasal delivery of targeted polyfunctional gold–iron oxide nanoparticles loaded with therapeutic microRNAs for combined theranostic multimodality imaging and presensitization of glioblastoma to temozolomide. Biomaterials (2019) 218:119342. doi:10.1016/j.biomaterials.2019.119342
232. Costa, PM, Cardoso, AL, Custódia, C, Cunha, P, Pereira De Almeida, L, and Pedroso De Lima, MC. MiRNA-21 silencing mediated by tumor-targeted nanoparticles combined with sunitinib: A new multimodal gene therapy approach for glioblastoma. J Controlled Release (2015) 207:31–9. doi:10.1016/j.jconrel.2015.04.002
233. Dhuri, K, Vyas, RN, Blumenfeld, L, Verma, R, and Bahal, R. Nanoparticle delivered anti-mir-141-3p for stroke therapy. Cells (2021) 10(5):1011. doi:10.3390/cells10051011
234. Chivero, ET, Liao, K, Niu, F, Tripathi, A, Tian, C, Buch, S, et al. Engineered extracellular vesicles loaded with miR-124 attenuate cocaine-mediated activation of microglia. Front Cel Dev. Biol. (2020) 8:573. doi:10.3389/fcell.2020.00573
235. Zhou, Y, Zhu, F, Liu, Y, Zheng, M, Wang, Y, Zhang, D, et al. Blood-brain barrier-penetrating siRNA nanomedicine for Alzheimer’s disease therapy. Sci Adv (2020) 6(41):7031. doi:10.1126/sciadv.abc7031
236. Grafals-Ruiz, N, Rios-Vicil, CI, Lozada-Delgado, EL, Quiñones-Díaz, BI, Noriega-Rivera, RA, Martínez-Zayas, G, et al. Brain targeted gold liposomes improve rnai delivery for glioblastoma. Int J Nanomedicine (2020) 15:2809–28. doi:10.2147/IJN.S241055
237. Conceição, M, Mendonça, L, Nóbrega, C, Gomes, C, Costa, P, Hirai, H, et al. Intravenous administration of brain-targeted stable nucleic acid lipid particles alleviates Machado-Joseph disease neurological phenotype. Biomaterials (2016) 82:124–37. doi:10.1016/j.biomaterials.2015.12.021
238. Alvarez-Erviti, L, Seow, Y, Yin, H, Betts, C, Lakhal, S, and Wood, MJA. Delivery of siRNA to the mouse brain by systemic injection of targeted exosomes. Nat Biotechnol (2011) 29(4):341–5. doi:10.1038/nbt.1807
239. Heidel, JD, Yu, Z, Liu, JYC, Rele, SM, Liang, Y, Zeidan, RK, et al. Administration in non-human primates of escalating intravenous doses of targeted nanoparticles containing ribonucleotide reductase subunit M2 siRNA. Proc Natl Acad Sci U S A (2007) 104(14):5715–21. doi:10.1073/pnas.0701458104
240. Yuan, Z, Zhao, L, Zhang, Y, Li, S, Pan, B, Hua, L, et al. Inhibition of glioma growth by a GOLPH3 siRNA-loaded cationic liposomes. J Neurooncol (2018) 140(2):249–60. doi:10.1007/s11060-018-2966-6
241. Ye, C, Pan, B, Xu, H, Zhao, Z, Shen, J, Lu, J, et al. Co-delivery of GOLPH3 siRNA and gefitinib by cationic lipid-PLGA nanoparticles improves EGFR-targeted therapy for glioma. J Mol Med (2019) 97(11):1575–88. doi:10.1007/s00109-019-01843-4
242. Chen, L, Watson, C, Morsch, M, Cole, NJ, Chung, RS, Saunders, DN, et al. Improving the delivery of SOD1 antisense oligonucleotides to motor neurons using calcium phosphate-lipid nanoparticles. Front Neurosci (2017) 11(AUG):476. doi:10.3389/fnins.2017.00476
243. Dowdy, SF. Overcoming cellular barriers for RNA therapeutics. Nat Biotechnol (2017) 35:222–9. doi:10.1038/nbt.3802
244. Yamada, Y. Nucleic acid drugs—current status, issues, and expectations for exosomes. Cancers (2021) 13:5002. doi:10.3390/cancers13195002
245. Kilikevicius, A, Meister, G, and Corey, DR. Reexamining assumptions about miRNA-guided gene silencing. Nucleic Acids Res (2022) 50:617–34. doi:10.1093/nar/gkab1256
246. Alles, J, Fehlmann, T, Fischer, U, Backes, C, Galata, V, Minet, M, et al. An estimate of the total number of true human miRNAs. Nucleic Acids Res (2019) 47(7):3353–64. doi:10.1093/nar/gkz097
247. Diener, C, Keller, A, and Meese, E. Emerging concepts of miRNA therapeutics: From cells to clinic. Trends Genet (2022) 38:613–26. doi:10.1016/j.tig.2022.02.006
248. Kim, M, Lee, Y, Lee, M, Yoo, J, and Kim, JS. Sequence-dependent twist-bend coupling in DNA minicircles. Nanoscale (2021) 13(33):20186–96. doi:10.1039/d1nr04672a
249. Wang, K, Kumar, US, Sadeghipour, N, Massoud, TF, and Paulmurugan, R. A microfluidics-based scalable approach to generate extracellular vesicles with enhanced therapeutic MicroRNA loading for intranasal delivery to mouse glioblastomas. ACS Nano (2021) 15(11):18327–46. doi:10.1021/acsnano.1c07587
250. Yang, YL, Zhang, XY, Wu, SW, Zhang, R, Zhou, BL, Zhang, XY, et al. Enhanced nose-to-brain delivery of siRNA using hyaluronan-enveloped nanomicelles for glioma therapy. J Controlled Release (2022) 342:66–80. doi:10.1016/j.jconrel.2021.12.034
251. Hao, R, Sun, B, Yang, L, Ma, C, and Li, S. RVG29-modified microRNA-loaded nanoparticles improve ischemic brain injury by nasal delivery. Drug Deliv (2020) 27(1):772–81. doi:10.1080/10717544.2020.1760960
252. Rassu, G, Soddu, E, Posadino, AM, Pintus, G, Sarmento, B, Giunchedi, P, et al. Nose-to-brain delivery of BACE1 siRNA loaded in solid lipid nanoparticles for Alzheimer’s therapy. Colloids Surf B Biointerfaces (2017) 152:296–301. doi:10.1016/j.colsurfb.2017.01.031
253. Borgonetti, V, and Galeotti, N. Intranasal delivery of an antisense oligonucleotide to the RNA-binding protein HuR relieves nerve injury-induced neuropathic pain. Pain (2021) 162(5):1500–10. doi:10.1097/j.pain.0000000000002154
254. Holm, A, Hansen, SN, Klitgaard, H, and Kauppinen, S. Clinical advances of RNA therapeutics for treatment of neurological and neuromuscular diseases. RNA Biol (2022) 19(1):594–608. doi:10.1080/15476286.2022.2066334
255. Kasina, V, Mownn, RJ, Bahal, R, and Sartor, GC. Nanoparticle delivery systems for substance use disorder. Neuropsychopharmacology (2022) 47:1431–9. doi:10.1038/s41386-022-01311-7
256. Shabanpoor, F, Hammond, SM, Abendroth, F, Hazell, G, Wood, MJA, and Gait, MJ. Identification of a peptide for systemic brain delivery of a morpholino oligonucleotide in mouse models of spinal muscular atrophy. Nucleic Acid Ther (2017) 27(3):130–43. doi:10.1089/nat.2016.0652
257. Kim, G, Kim, M, Lee, Y, Byun, JW, Hwang, DW, and Lee, M. Systemic delivery of microRNA-21 antisense oligonucleotides to the brain using T7-peptide decorated exosomes. J Controlled Release (2020) 317:273–81. doi:10.1016/j.jconrel.2019.11.009
258. Mizuo, K, and Okazaki, S. Acute ethanol administration increases mir-124 expression via histone acetylation in the brain. J Alcohol Drug Depend (2016) 04(02). doi:10.4172/2329-6488.1000232
259. Sadakierska-Chudy, A, Frankowska, M, Miszkiel, J, Wydra, K, Jastrzębska, J, and Filip, M. Prolonged induction of miR-212/132 and REST expression in rat striatum following cocaine self-administration. Mol Neurobiol (2017) 54(3):2241–54. doi:10.1007/s12035-016-9817-2
260. Nunez, YO, Truitt, JM, Gorini, G, Ponomareva, ON, Blednov, YA, Harris, RA, et al. Positively correlated miRNA-mRNA regulatory networks in mouse frontal cortex during early stages of alcohol dependence. BMC Genomics (2013) 14(1):725. doi:10.1186/1471-2164-14-725
261. Morgan, BS, Sanaba, BG, Donlic, A, Karloff, DB, Forte, JE, Zhang, Y, et al. R-BIND: An interactive database for exploring and developing RNA-targeted chemical probes. ACS Chem Biol (2019) 14(12):2691–700. doi:10.1021/acschembio.9b00631
262. Disney, MD, Winkelsas, AM, Velagapudi, SP, Southern, M, Fallahi, M, and Childs-Disney, JL. Inforna 2.0: A platform for the sequence-based design of small molecules targeting structured RNAs. ACS Chem Biol (2016) 11(6):1720–8. doi:10.1021/acschembio.6b00001
263. Turowski, P, and Kenny, BA. The blood-brain barrier and methamphetamine: Open sesame? Front Neurosci (2015) 9:156. doi:10.3389/fnins.2015.00156
264. Hong, DS, Kang, YK, Borad, M, Sachdev, J, Ejadi, S, Lim, HY, et al. Phase 1 study of MRX34, a liposomal miR-34a mimic, in patients with advanced solid tumours. Br J Cancer (2020) 122(11):1630–7. doi:10.1038/s41416-020-0802-1
265. Liu, C, Kelnar, K, Liu, B, Chen, X, Calhoun-Davis, T, Li, H, et al. The microRNA miR-34a inhibits prostate cancer stem cells and metastasis by directly repressing CD44. Nat Med (2011) 17(2):211–5. doi:10.1038/nm.2284
266. Bader, AG. MiR-34 - a microRNA replacement therapy is headed to the clinic. Front Genet (2012) 3(JUL):120. doi:10.3389/fgene.2012.00120
267. Kelnar, K, and Bader, AG. A qRT-PCR method for determining the biodistribution profi le of a miR-34a mimic. Methods Mol Biol (2015) 1317:125–33. doi:10.1007/978-1-4939-2727-2_8
268. Moulder, SL, Symmans, WF, Booser, DJ, Madden, TL, Lipsanen, C, Yuan, L, et al. Phase I/II study of G3139 (Bcl-2 antisense oligonucleotide) in combination with doxorubicin and docetaxel in breast cancer. Clin Cancer Res (2008) 14(23):7909–16. doi:10.1158/1078-0432.CCR-08-1104
269. Sternberg, CN, Dumez, H, van Poppel, H, Skoneczna, I, Sella, A, Daugaard, G, et al. Docetaxel plus oblimersen sodium (Bcl-2 antisense oligonucleotide): An EORTC multicenter, randomized phase II study in patients with castration-resistant prostate cancer. Ann Oncol (2009) 20(7):1264–9. doi:10.1093/annonc/mdn784
270. Anderson, EM, Miller, P, Ilsley, D, Marshall, W, Khvorova, A, Stein, CA, et al. Gene profiling study of G3139- and Bcl-2-targeting siRNAs identifies a unique G3139 molecular signature. Cancer Gene Ther (2006) 13(4):406–14. doi:10.1038/sj.cgt.7700901
271. Lai, JC, Tan, W, Benimetskaya, L, Miller, P, Colombini, M, and Stein, CA. A pharmacologic target of G3139 in melanoma cells may be the mitochondrial VDAC. Proc Natl Acad Sci U S A (2006) 103(19):7494–9. doi:10.1073/pnas.0602217103
272. Pisano, M, Baldinu, P, Sini, MC, Ascierto, PA, Tanda, F, and Palmieri, G. Targeting Bcl-2 protein in treatment of melanoma still requires further clarifications. Ann Oncol (2008) 19:2092–3. doi:10.1093/annonc/mdn672
273. Sago, CD, Lokugamage, MP, Paunovska, K, Vanover, DA, Monaco, CM, Shah, NN, et al. High-throughput in vivo screen of functional mRNA delivery identifies nanoparticles for endothelial cell gene editing. Proc Natl Acad Sci U S A (2018) 115(42):E9944–E9952. doi:10.1073/pnas.1811276115
274. Shu, J, Xia, Z, Li, L, Liang, ET, Slipek, N, Shen, D, et al. Dose-dependent differential mRNA target selection and regulation by let-7a-7f and miR-17-92 cluster microRNAs. RNA Biol (2012) 9(10):1275–87. doi:10.4161/rna.21998
Keywords: microRNA, substance use disorder, noncoding RNA, lncRNA, SMIRNA, circRNA
Citation: Seyednejad SA and Sartor GC (2022) Noncoding RNA therapeutics for substance use disorder. Adv. Drug. Alco. Res. 2:10807. doi: 10.3389/adar.2022.10807
Received: 29 July 2022; Accepted: 14 November 2022;
Published: 20 December 2022.
Edited by:
Stephanie Sillivan, Temple University, United StatesReviewed by:
Zheng-Xiong Xi, National Institute on Drug Abuse (NIH), United StatesPeter Hamilton, Virginia Commonwealth University, United States
Copyright © 2022 Seyednejad and Sartor. This is an open-access article distributed under the terms of the Creative Commons Attribution License (CC BY). The use, distribution or reproduction in other forums is permitted, provided the original author(s) and the copyright owner(s) are credited and that the original publication in this journal is cited, in accordance with accepted academic practice. No use, distribution or reproduction is permitted which does not comply with these terms.
*Correspondence: Gregory C. Sartor, R3JlZ29yeS5zYXJ0b3JAdWNvbm4uZWR1