- 1Institute of Virology, Biomedical Research Center of the Slovak Academy of Sciences, Bratislava, Slovakia
- 2Biotechnological and Analytical Laboratories, Biomedical Research Center of the Slovak Academy of Sciences, Sarišské Michal’any, Slovakia
- 3Institute of Materials and Machine Mechanics, Slovak Academy of Sciences, Bratislava, Slovakia
The current pandemic of SARS-CoV-2 coronavirus infection has highlighted the importance of control measures to combat infections caused by airborne pathogens. Non-specific actions include various means of microorganism inactivation by chemical or physical methods targeting structural components of a given pathogen. Exposure of viruses and bacteria to elevated temperatures is one of the effective methods for discharging their harmful potential. Using a model of the Human Adenovirus 5 exposed to elevated temperatures with subsequent titration of the virus in vitro in A549 cells, we showed a dramatic decrease of the virus titer after heat treatment for 5 s at temperatures over 100°C. To verify the potential of the heat inactivation in a closed environment, the prototype of a high-capacity pathogen-cleaning device was constructed and tested. Application of the device for 2 h at an airflow rate of 900 m3/h in a room of 226 m3 reduced the total number of microorganisms in the air by more than 50% at all collection sites in the room.
The COVID-19 pandemic has been devastating the planet for more than 2 years in several consecutive waves, causing enormous damage to public health and social life with an enormous impact on the world economy. Thanks to the rapid development of effective vaccines, it was possible to successfully combat the pandemic (Hossaini Alhashemi et al., 2023). However, the causative agent of COVID-19, the Severe Acute Respiratory Syndrome Coronavirus 2 (SARS-CoV-2), continues to mutate into new variants that have increased potential to break the vaccination barrier (Hillary and Ceasar, 2023). The most effective way to combat SARS-CoV-2 is to combine vaccination while adhering to non-intervention measures, especially in public places with an increased concentration of people. In addition to standard approaches to personal protection such as wearing masks and gloves, keeping personal hygiene and keeping distance, frequent ventilation and fresh air exchange play an important role (Juneau et al., 2022). SARS-CoV-2 belongs to a group of viruses that spread effectively in the environment through tiny droplets (less than 5 µm) in the form of an aerosol, which enables the virus to spread effectively in the population (Chen et al., 2021; Wang et al., 2021). Therefore, to minimize the risk of infection in public places, it is very important to ensure sufficient air exchange and cleaning from viruses and other microorganisms in public areas such as in hospitals, theaters, cinemas or fitness centers. The idea of the inactivation of microorganisms in the air is not new and there were several devices constructed that usually operate either on the principle of mechanical separation using suitable filters, UV radiation or chemically using various disinfectants. Each of these methods has its advantages and disadvantages, but their use in large public places is relatively limited. In addition to the above-mentioned approaches, viruses, including adenoviruses and coronaviruses, as well as microorganisms are sensitive to the elevated temperature, at which they are inactivated and lose their infectivity, therefore treatment of viruses by heat appears to be a good alternative (Maheshwari et al., 2004; Chan et al., 2011; Kampf et al., 2020). In laboratory conditions, high temperature as a means of virus inactivation is a well-established and reliable approach. In general, there is a lot of scientific work that has studied the thermal inactivation of viruses at temperatures up to 100°C including SARS-CoV-2, for which complete inactivation at 70°C for 3 min has been recommended (Charm et al., 1992; Abraham et al., 2020; Batéjat et al., 2021). As the temperature decreases, the time for the virus inactivation increases. However, there are only scarce data on the thermal resistance of viruses at temperatures above 100°C (Yu et al., 2020).
In our work, we primarily focused on the issue of virus survival at high temperatures as a function of time and temperature. For the initial study, we used the model of human adenovirus type 5 (HAdV-5). The ultimate aim of the work was to define the conditions of virus inactivation after its flow-through exposure in a high-capacity air disinfection system operating on the principle of high temperature (above 100°C). For that purpose, a model situation has been designed to enable high-temperature setting. Small cups of a defined shape and volume were prepared from aluminum foil. Before the experiment, cups were exposed to UV radiation for 15 min for their sterilization. In the first experiments, suitable time intervals and volumes of medium were selected with the aim to minimize loss by evaporation. Subsequently, 50 µL of DMEM culture medium containing HAdV-5 at a concentration of 1 × 107 PFU/mL were added to the aluminum cup. The sample was exposed to the temperature ranging from 60°C up to 180°C for 5 s, utilizing an induction plate. Then the samples were immediately removed from the plate, cooled by adding 1 mL of DMEM culture medium and incubated for 5 min at room temperature. Subsequently, all samples were evaluated by the standard plaque titration method in A549 cells. Plaque-counting was accomplished 7 days post-infection in cell monolayers stained with 4% formaldehyde and crystal violet. As expected, viral titers decreased with increasing temperature (Figure 1). We were not able to detect any viable viral particles in the medium heated to the temperature of 140°C. This suggests that direct exposure of virus to a temperature above 140°C for 5 s is sufficient for its complete inactivation in laboratory conditions.
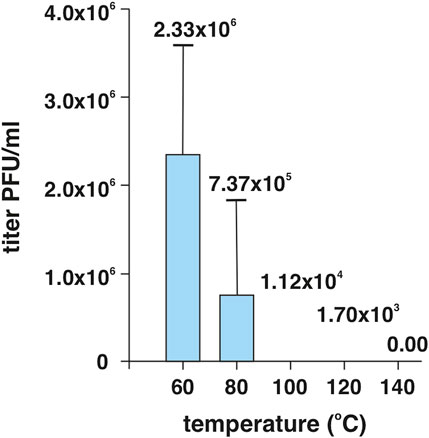
FIGURE 1. Temperature-dependent survival of HAdV-5 viral particles in the DMEM culture medium. The virus at an initial dose of 1 × 107 PFU/mL was exposed to heat for 5 s, then cooled and subsequently subjected to titration on A459 human lung carcinoma cells. The experiment was performed in triplicates.
From the practical point of view, the aim of this primary study was to determine the minimum temperature, at which the virus is completely inactivated, to enable set-up of technical requirements and parameters to design and construct a high-capacity air-cleaning device. In the next step of the study, the functional model of the high-capacity pathogen-inactivating device has been designed and further tested in semi-operation conditions. In the designed prototype (subject to IP protection) the contaminated air is flowing through multiple heat exchangers into the inactivation chamber, where it remains for the required inactivation time. Subsequently, the already decontaminated air comes out through the opposite side of the exchanger, while it is cooled down to the original room temperature. In this way, the sterilized air retains its original moisture and composition. The advantage of the designed solution is the high flow capacity of the device with minimal energy requirements, because the contaminated air is mostly warmed up by heat that is taken from the air during the reverse flow from the inactivation chamber. The used device made it possible to achieve an airflow of 100 L/s, i.e., it was able to process a volume of 360 m3 per hour. Thermal air treatment is harmless to humans and animals, does not produce harmful substances, does not create any waste, nor does it require any consumables. Therefore, the device can work in a continuous mode without limiting the normal operation in the room. From our initial experiments, we postulate that a temperature in the range of 120°C–140°C should be suitable for use in the model air cleaner. The prototype TRL 3-4 device was initially tested for physical parameters such as performance, energy consumption and temperature distribution. The experimental setup for the prototype testing in semi-operation conditions included its placement in a technical room with a volume of 226 m3, collection of air samples at eight different sampling points and subsequent evaluation of microbial load in individual air samples at different time points after the device operation. This approach with a focus on natural microbial load was chosen because in these conditions, it was not possible to directly introduce infectious viruses. The air collection points were in the close vicinity of the device outlet (No. 3), in close distance within 2 m from the device (Nos 1, 2, 4) and more than 3 m from the air cleaner (Nos 5, 6, 7, 8) (Figure 2). There were four time points of the air samples collection (before the start of the experiment and after 1, 2 or 3 h of the device operation); air sampling was performed using MAS-100 NT Microbial Air Sampler (Merck), and the samples were plated on trypton-soya agar plates that support the growth of a wide variety of microorganisms, including most of bacteria and yeasts. Thus, the results obtained after incubation of plates at 37°C for 7 days represented the number of all growing microorganisms per m3 without their further specification. The data outlined in Table 1 clearly demonstrate that the reduction of circulating live microorganisms can go to more than 50% of the original load at all sample collection points after 2 h of high-capacity air-cleaner device operation. However, the time interval of 5 s exposure to metal plates in heat exchanger can be easily extended, thereby increasing the efficiency of thermal inactivation of air actively flowing through the device.
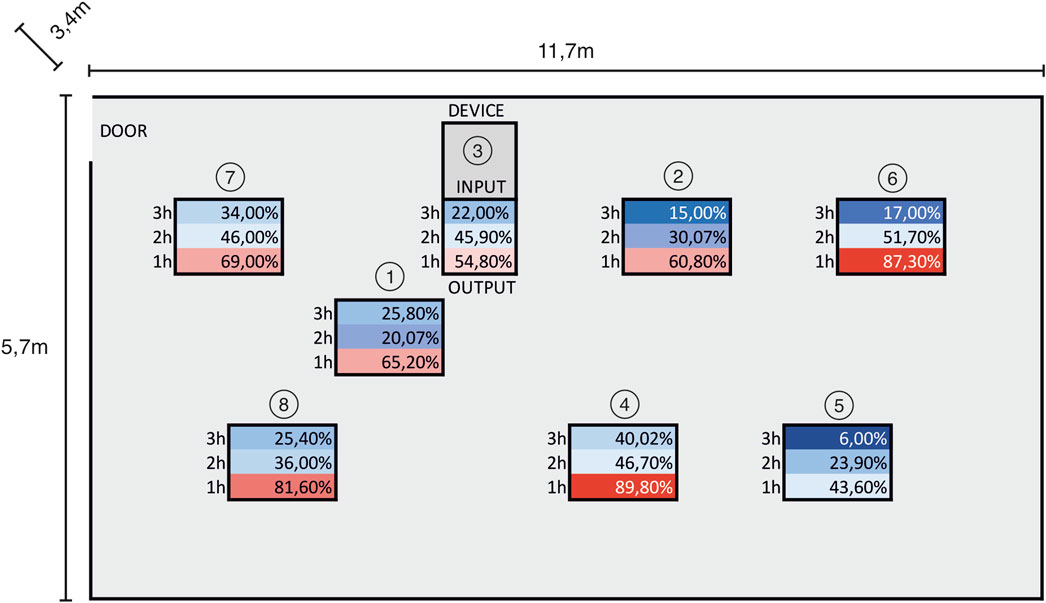
FIGURE 2. Collection sites and % of remaining colonies at different time points after operation of the high-capacity air cleaner.
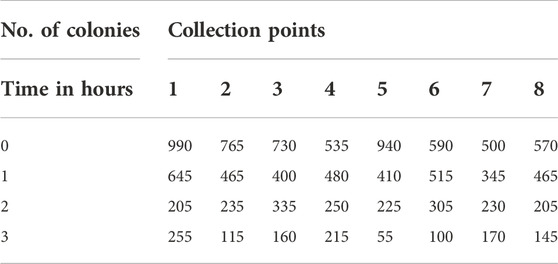
TABLE 1. Number of microorganisms grown on TSA plates per m3 of room space (colonies count) at different time points.
Although we used a different approach for the device setup, our results are in close agreement with Yu et al. (2020) study where the authors found that 99.8% of the aerosolized SARS-CoV-2 were killed by a single pass through a Ni-foam-based filter heated up to 200°C. In a different work, the screening of sterilization temperatures showed that it should be higher than 100°C–120°C to achieve a high degree of virus inactivation. In this work, a temperature of 150°C was adopted in most of the simulations (Busto et al., 2022), which is consistent with our findings.
The current pandemic has once again drawn attention to the issue of efficient, effective and cheap air conditioning and heat-inactivating device in order to clean the internal public environment of dangerous pathogens. Our data presented here provide a good basis for such an approach in the fight not only against the COVID-19 pandemic but also against other airborne infections, particularly at sites of their high potential accumulation, such as in hospitals or other densely occupied public spaces.
Data availability statement
The original contributions presented in the study are included in the article/supplementary material, further inquiries can be directed to the corresponding author.
Author contributions
All authors listed have made a substantial, direct, and intellectual contribution to the work and approved it for publication.
Acknowledgments
This work was funded by the Slovak Research and Development Agency (SARSkill project, APVV-PP-COVID-20-0098). This publication was created thanks to support under the Operational Programme Integrated Infrastructure for the project–IMTS code: 313011ASU8 (EVAgoEAST) co-financed by the European Regional Development Fund.
Conflict of interest
The authors declare that the research was conducted in the absence of any commercial or financial relationships that could be construed as a potential conflict of interest.
Abbreviations
A549, human lung adenocarcinoma cell line; COVID-19, Coronavirus disease 19; DMEM, Dulbecco’s Modified Eagle’s medium; HAdV-5, human adenovirus type 5; PFU, plaque forming unit; SARS-CoV-2, Severe Acute Respiratory Syndrome Coronavirus 2.
References
Abraham, J. P., Plourde, B. D., and Cheng, L. (2020). Using heat to kill SARS-CoV-2. Rev. Med. Virol. 30 (5), e2115. doi:10.1002/rmv.2115
Batéjat, C., Grassin, Q., Manuguerra, J. C., and Leclercq, I. (2021). Heat inactivation of the severe acute respiratory syndrome coronavirus 2. J. Biosaf. Biosecur 3 (1), 1–3. doi:10.1016/j.jobb.2020.12.001
Busto, M., Tarifa, E. E., Cristaldi, M., Badano, J. M., and Vera, C. R. (2022). Simulation of thermal sanitization of air with heat recovery as applied to airborne pathogen deactivation. Int. J. Environ. Sci. Technol. (Tehran) 19 (12), 11685–11698. doi:10.1007/s13762-022-03948-9
Chan, K. H., Peiris, J. S., Lam, S. Y., Poon, L. L., Yuen, K. Y., and Seto, W. H. (2011). The effects of temperature and relative humidity on the viability of the SARS coronavirus. Adv. Virol. 2011, 734690. doi:10.1155/2011/734690
Charm, S. E., Landau, S., Williams, B., Horowitz, B., Prince, A. M., and Pascual, D. (1992). High-temperature short-time heat inactivation of HIV and other viruses in human blood plasma. Vox Sang. 62 (1), 12–20. doi:10.1111/j.1423-0410.1992.tb01160.x
Chen, P. Z., Bobrovitz, N., Premji, Z., Koopmans, M., Fisman, D. N., and Gu, F. X. (2021). Heterogeneity in transmissibility and shedding SARS-CoV-2 via droplets and aerosols. Elife 10, e65774. doi:10.7554/eLife.65774
Hillary, V. E., and Ceasar, S. A. (2023). An update on COVID-19: SARS-CoV-2 variants, antiviral drugs, and vaccines. Heliyon 9 (3), e13952. doi:10.1016/j.heliyon.2023.e13952
Hossaini Alhashemi, S., Ahmadi, F., and Dehshahri, A. (2023). Lessons learned from COVID-19 pandemic: Vaccine platform is a key player. Process Biochem. 124, 269–279. doi:10.1016/j.procbio.2022.12.002
Juneau, C. E., Pueyo, T., Bell, M., Gee, G., Collazzo, P., and Potvin, L. (2022). Lessons from past pandemics: A systematic review of evidence-based, cost-effective interventions to suppress COVID-19. Syst. Rev. 11 (1), 90. doi:10.1186/s13643-022-01958-9
Kampf, G., Voss, A., and Scheithauer, S. (2020). Inactivation of coronaviruses by heat. J. Hosp. Infect. 105 (2), 348–349. doi:10.1016/j.jhin.2020.03.025
Maheshwari, G., Jannat, R., McCormick, L., and Hsu, D. (2004). Thermal inactivation of adenovirus type 5. J. Virol. Methods 118 (2), 141–146. doi:10.1016/j.jviromet.2004.02.003
Wang, C. C., Prather, K. A., Sznitman, J., Jimenez, J. L., Lakdawala, S. S., Tufekci, Z., et al. (2021). Airborne transmission of respiratory viruses. Science 373 (6558), eabd9149. doi:10.1126/science.abd9149
Keywords: heat inactivation, airborne pathogen, SARS-CoV-2, COVID-19, human adenovirus 5
Citation: Slavikova M, Strukova J, Klempa B, Simancik F, Pastorekova S, Zelnik V and Kopacek J (2023) Heat inactivation by high temperature as an approach to combat infections caused by airborne pathogens. Acta Virol. 67:11640. doi: 10.3389/av.2023.11640
Received: 04 April 2023; Accepted: 26 May 2023;
Published: 27 June 2023.
Edited by:
Katarina Polcicova, Slovak Academy of Sciences, SlovakiaCopyright © 2023 Slavikova, Strukova, Klempa, Simancik, Pastorekova, Zelnik and Kopacek. This is an open-access article distributed under the terms of the Creative Commons Attribution License (CC BY). The use, distribution or reproduction in other forums is permitted, provided the original author(s) and the copyright owner(s) are credited and that the original publication in this journal is cited, in accordance with accepted academic practice. No use, distribution or reproduction is permitted which does not comply with these terms.
*Correspondence: Juraj Kopacek, anVyYWoua29wYWNla0BzYXZiYS5zaw==