- 1Institute of Health Sciences, Marmara University, Istanbul, Türkiye
- 2University of Health Sciences Kartal Koşuyolu High Speciality Educational and Research Hospital, Istanbul, Türkiye
- 3Department of Basic Pharmaceutical Sciences, Faculty of Pharmacy, Marmara University, Istanbul, Türkiye
Influenza A viruses are enveloped viruses with a genome of eight single-stranded negative-sense RNA molecules. In virions, RNA segments are found as vRNPs associated with NP proteins. The RdRp enzyme, which catalyzes the replication/transcription of the viral genome, is carried as attached to vRNPs. In this study, it was demonstrated that the PA subunit of the viral RdRp interacts with β-actin proteins by the yeast two-hybrid assay. It was shown that the amino-terminal domains of the β-actin protein bind to the carboxy-terminal moiety of the viral PA protein in the mammalian cells. The results were supported by in silico analysis. Over-expression of the β-actin protein was found to have a negative effect on the viral RdRp activity in mini-replicon, but its mechanism of action has remained unknown. The results suggest that the interaction of β-actin and PA protein, a component of vRNPs, may have a role in the intracellular trafficking of the influenza vRNPs and/or viral transcription.
Introduction
The influenza A virus is an enveloped virus with a single-stranded negative polarity RNA genome consisting of eight segments. Viral RNAs form complexes with nucleoproteins (NP) called viral ribonucleoprotein particles (vRNP) (Couch, 1996). The RNA-dependent RNA polymerase (RdRp) enzyme, which catalyzes the replication and transcription of the viral genome in infected cells, is a heterotrimeric molecule consisting of PB2, PB1, and PA proteins encoded by the first three largest RNA segments (Te Velthuis and Fodor, 2016). The viral RdRp enzymes are found attached to the vRNPs in the virions (Pflug et al., 2017). Due to their limited genetic coding capacity, viruses completely depend on the host cellular transcription and translation machinery for their survival. Therefore, from the first stage of the virus infection until the assembly of the mature virion, many cellular and viral proteins interact with each other directly or indirectly. In these interactions, cellular proteins may be involved in the entry and release of the virions into the cell (Dou et al., 2018) as well as in intracellular trafficking of viral components (York and Fodor, 2013) or may inhibit viral replication (Hoang et al., 2021). Although the main functions of influenza RdRp are replication and transcription, the subunits of the enzyme interact with some cellular proteins and affect the course of the viral replication.
The PA subunit of the RdRp has been found to play an important role in the virus replication cycle and adaptation to the host cells (Mehle et al., 2012; Sun et al., 2014). It is known that the PA protein is involved in an interaction network with many cellular proteins such as the human cyclin-dependent kinase inhibitor p21 (p21) (Ma et al., 2022); the HCLS1-associated protein X-1 (HAX1), a cellular protein with an anti-apoptotic effect (Hsu et al., 2013); and the nucleolin (NCL), a major nucleolar phosphoprotein of growing cells, Gao et al. (2018). Although these data do not give an exact conclusion regarding the interaction models and mechanism of action between the PA and cellular proteins, they show that this protein is a multifunctional protein performing different roles in addition to its basic transcription/replication function. In this study, the interaction between the influenza virus PA and human β-actin protein, which was found to bind to the PA protein used as bait in yeast two-hybrid assay screening, was evaluated in mammalian cells.
Actins are evolutionarily well conserved proteins in eukaryotic cells. Although the vast majority of β-actin is found in the cytoplasm, some actin proteins in the nucleus are also present. The nucleocytoplasmic transport of β-actin is regulated by importin-9 and exportin-6 (Stuven et al., 2003; Dopie et al., 2012). The cytoplasmic β-actin is basically responsible for the mechanical properties and shapes of cells and plays a crucial role in fundamental functions such as cell migration, phagocytosis, vesicular transport, and cell division (Bunnell et al., 2011). Furthermore, the nuclear actin protein has also attracted a lot of attention in terms of its function. It has been clearly demonstrated that actin and several actin-related proteins play crucial roles in various nuclear activities such as chromatin remodeling, RNA transcription, and the regulation of gene expressions (Zheng et al., 2009). Moreover, it has been shown that β-actin protein is also important for the replication of many viruses such as enterovirus 71 (EV71) (Lui et al., 2013), the swine fever virus (CSFV) (He et al., 2014), and the coronavirus infectious bronchitis virus (IBV) (Wang et al., 2009) and play a role in making the virus infection more efficient. Here, we shown that human β-actin interacts with the PA subunit of viral RdRP in both yeast and mammalian cells, suggesting that this protein may have a role in vRNP trafficking and/or viral transcription.
Materials and methods
Mammalian cells and maintenance
HeLa (human cervical cancer cells) and HEK293 (human embryonic kidney cells) cell lines were obtained from Department of Infection Biology, Faculty of Medicine, Tsukuba University. The cells were maintained in Dulbecco’s modified eagle medium (DMEM); supplemented with 10% fetal bovine serum (FCS), 2 mM L-glutamine, 100 U/mL penicillin, and 100 μg/mL streptomycin; and cultured under the standard culture conditions (37°C, 5% CO2, 95% relative humidity).
Yeast cells
The Saccharomyces cerevisiae strain PJ69-4A was used to detect the proteins interacting with the influenza A virus PA protein by the yeast two-hybrid assay [Genotype: MATa trp1-901 leu2-3, 112 ura3-52 his3-200 gal4 (deletion) gal80 (deletion) LYS2::GAL1-HIS3 GAL2-ADE2 met2::GAL7-lacZ]. The cells were grown in a YPAD (yeast extract- peptone-dextrose plus adenine) rich medium at 30°C at 175 rpm shaking and stored at −80°C in a YPD (yeast extract-peptone-dextrose) medium containing 20% glycerin.
Bacterial cells
Gene cloning and amplification of plasmid DNAs were performed with the Escherichia coli Mach1 (Invitrogen) strain. The cells were cultured in Luria-Bertani (LB) broth and Luria-Bertani Agar (LBA) media.
Viruses
The cells were infected with human influenza A/WSN/33-H1N1 (WSN) viruses. The viruses were grown in embryonated chicken eggs and/or MDCK cells. Virus titration was determined by a plaque forming assay (Turan et al., 1996).
Vector construction
The expression vectors encoding HA (YPYDVPDYA) tagged β-actin (hACTB) and β-actin variants with deleted regions corresponding to specific exons were generated by cloning the cDNA into the pCHA plasmid (Nagata et al., 1998). The plasmid DNA was digested with the EcoRV restriction enzyme (New England Biolabs, United States) and dephosphorylated with shrimp alkaline phosphatase (SAP) (Thermo Scientific, United States). β-actin open reading frame (ORF) was amplified with PCR over the cDNA library of HEK293 by using 5′-ATCATGGATGATGATATCGCCGC-3′ and 5′-ATCTAGAAGCATTTGCGGTGGAC-3′ primer pairs phosphorylated with T4 polynucleotide kinase (New England Biolabs, United States). PCR was performed as: 1 cycle “95°C, 3 min” initial denaturation; 30 cycles “95°C, 15 s; 56°C, 15 s; 68°C, 1 min”; and 68°C, 5 min final extension. The reaction was carried out with the high fidelity KOD DNA polymerase enzyme (Toyobo, Japan) which produces a blunt-end product. The PCR products were purified from agarose gel with a DNA agarose gel extraction kit (Qiagen, Germany) and ligated with the pCHA plasmid DNA in the frame of the HA-tag coding sequence by using T4 DNA ligase (Takara, Japan), and the resultant plasmid was designated as pCHA-ACTB. Plasmid vectors having a deletion of certain exons in the β-actin gene were derived from the pCHA-ACTB by inverse PCR. The primers were phosphorylated with T4-polynucleotide kinase. For the gene lacking the second and third exons (ACTBΔ2.3), ACTΔ2.3-For/Vec-Rev primers; for the gene lacking the third exon (ACTBΔ3), ACTΔ3-For/ACTΔ3-Rev primers; for the gene lacking the fifth and sixth exons (ACTBΔ5.6), Vec-For/ACTΔ5.6-Rev primers; and for the gene lacking the sixth exon (ACTBΔ6), Vec-For/ACTΔ6-Rev primers (Table 1) were used. The PCR was carried out with KOD DNA polymerase and conducted as: 1 cycle “95°C, 3 min” initial denaturation; 30 cycles “95°C, 20 s; 54°C, 15 s; 68°C, 5 min”; and 68°C, 10 min final extension. The PCR products were purified with a DNA agarose gel extraction kit and self-ligated with T4 DNA ligase. Ligated DNAs were transformed into competent E. coli cells, and the cells were grown on LBA (+amp) medium. Plasmid DNAs isolated from the colonies were checked by cutting with restriction endonuclease enzymes.
Yeast two-hybrid plasmid coding GAL4 binding domain (GAL4-BD)—viral PA fusion (BD-cPA) was constructed by cloning a 3′-half of the influenza A virus PA gene consisting of 1,074 nucleotides (cPA) into pGBD-C1 plasmid DNA (James et al., 1996). For this, the plasmid DNA was digested with EcoRI just after the GAL4-BD coding region, blunted with the Klenow enzyme, and then dephosphorylated with SAP. The cPA ORF carrying the linker sequence (ggaggatctgga/Gly-Gly-Ser-Gly) at 5′-end was amplified with PCR by using the pCAGGS-PA plasmid DNA (Pham et al., 2018) as a template and phosphorylated cPA-For/PA-Rev primers (Table 1) and purified with a DNA gel extraction kit. A pGBD-cPA bait plasmid was obtained with ligation of the cPA DNA fragment and linearized pGBD-C1 plasmid.
A pCAGGS-GSTnls plasmid (Sugiyama et al., 2015) was used to obtain the plasmid vectors encoding GST-tagged β-actin (GST.ACTB) and the carboxy-terminal half of the influenza PA (GST.cPA) fusion proteins. The pCAGGS-GSTnls was linearized by inverse PCR using the pCA-Vec-For/GST (GGSG)-Rev primers (Table 1) and ligated with PCR-amplified β-actin or cPA cDNA. The resultant plasmids were named pCAGGS-GST.ACTB and pCAGGS-GST.cPA.
The plasmid vectors used in the study and the proteins encoded by these vectors are given in Table 2. The nucleotide sequences of all genes cloned into the plasmids were confirmed by the Sanger DNA sequencing method.
Yeast two-hybrid cDNA library
The cDNA library constructed by cloning human embryonic kidney cells (HEK293) cDNA immediately after the sequence of yeast GAL4 activation domain (GAL4-AD) in pACT2 plasmid (pACT2-cDNAn) was purchased as transformed in E. coli cells (Clontech #638826). The cells were grown on LB agar (+amp) plates according to the manufacturer’s instructions, and the plasmid DNA was isolated.
Yeast two-hybrid assay
The yeast two-hybrid (Y2H) assay was performed as previously described (Senbas-Akyazi et al., 2020; Pirincal and Turan, 2021), taking into account the manufacturer’s instructions. Briefly, a small-scale yeast culture (5 mL) was prepared in the YPAD medium and transformed with the pGBD-cPA plasmid DNA coding the bait protein (GAl4-BD.cPA) by the lithium acetate/polyethylene glycol (LiAc/PEG) method (Gietz and Schiestl, 2007). Transformants were selected on a yeast synthetic drop-out (SD) agar medium (without Trp). One of the colonies harboring the bait plasmid was grown in the YPAD medium, transformed with the Y2H Matchmaker cDNA library, and seeded on SD agar plates (without Her, Leu, Ade, and Trp) for selection of positive colonies. The relative β-galactosidase activities of the yeast colonies grown on the selective media were measured by using an o-nitrophenyl-β-D-galactopyranoside (ONPG) substrate (Senbas-Akyazi et al., 2020). The plasmids carrying cDNA were isolated from the colonies having high β-galactosidase activity with a yeast plasmid DNA extraction kit (Bio Basic, Canada). The cDNAs carried on the plasmids were amplified with PCR using the oligonucleotide primers 5′-AATACCACTACAATGGATGATGT-3′ and 5′-CCAAGATTGAAACTTAGAGGAGT-3’. The PCR products were purified with a gel extraction kit, and the sequence of the cDNAs was defined with Sanger sequencing. The cDNAs were identified with the basic local alignment search tool (BLAST).
Plasmid transfection
HEK293 and HeLa cells were transiently transfected with the plasmid DNA using polyethyleneimine (PEI) (Longo et al., 2013). The cells were seeded in a DMEM complete medium in multi-well plates (1 × 105 HEK293 cells/well or 5 × 104 HeLa cells/well in 24-well plate; 2 × 105 HEK293 cells/well or 1 × 105 HeLa cells/well in 12-well plate) or a 6 cm Petri dish (1 × 106 HEK293 cells) and incubated overnight under standard culture conditions. An average of 0.25–0.5 µg/well (in 24-well plate), 0.5–1 µg/well (in 12-well plate), or 2.5–5 µg of plasmid DNA (6 cm Petri dish) were used for transfection of the cells. Plasmid DNAs were diluted to 15 ng/μL in OPTİ-MEM (Thermo Scientific, Gibbco, United States). The 20 μg/μL PEI solution in deionized distilled water was also diluted in OPTİ-MEM to a 40–50 ng/μL final concentration. Diluted DNA and PEI solutions were mixed in equal volumes, incubated at room temperature for 5–10 min, and added to the cell cultures. The cultures were incubated for 10 h under standard culture conditions, and then the media were replaced with fresh media. The cells were harvested 45–48 h after transfection.
Immunoblotting
The proteins were expressed in transfected and/or infected cells or precipitated with glutathione-Sepharose beads (GE-Healthcare, CL-4B) and protein A-Sepharose (GE-Healthcare, CL-4B) and were analyzed by immunoblotting. The proteins were separated with sodium dodecyl sulfate-polyacrylamide gel electrophoresis (SDS-PAGE) and transferred to PVDF membranes. The membranes were blocked in 5% skim milk in tris-buffered saline (TBS) (137 mM NaCl, 20 mM Tris, pH: 7.4) with 0.1% Tween 20 (TBS-T) at room temperature for 60 min and subsequently treated with mouse anti-HA (Santa Cruz, sc-7392) (for HA-tagged β-actin proteins), mouse anti-actin (Abcam, ab11003) (for endogenous β-actin), rabbit anti-PA, or M1 rabbit antibodies [kindly provided by Dr. K. Nagata (Kawaguchi et al., 2005; Takizawa et al., 2006)] in skim milk at 4°C overnight. After washing thoroughly in TBS-T, the membranes were incubated with the goat anti-mouse IgG-HRP antibody (Invitrogen/Thermo: 31420) or anti-rabbit IgG-HRP antibody (Invitrogen/Thermo: 31423) diluted at a 1:5,000 in TBS for 30 min at room temperature. The membranes were washed with TBS-T/TBS and visualized with a gel imaging system (DNR Bio-Imaging system) using chemiluminescence substrate (H&C, RPN2232).
Immunofluorescence assay
The expression and intracellular localization of the proteins in transiently transfected HeLa cells were defined with an immunofluorescence assay. The cells were grown in 12-well plates on glass coverslips for 24 h and transfected with plasmid DNAs using PEI. After 45–48 h of transfection, the cells were fixed in 3% paraformaldehyde for 10 min, rinsed three times with phosphate buffered saline (PBS), and permeabilized with 0.1% NP-40. The cells were blocked in 1% skim milk in PBS for 30 min and treated with primary antibodies for 60 min at 1/250–1/500 dilution in the milk. Filamentous actin proteins in transiently transfected cells were labeled with Alexa 568-Phalloidin (Invitrogen, Thermo Fisher Scientific # A12380) at 1/200 dilution. After rinsing three times with PBS, the cells were blocked a second time in the milk for 20 min and treated with anti-mouse Alexa 488 (Abcam: ab150117) and/or anti-rabbit Alexa 568 secondary antibodies (Abcam: ab175471) at 1/300 dilution for 60 min. For visualization of the cell nuclei, DAPI (4′, 6-diamidino-2-phenylindole) was added to the antibody solutions at a concentration of 0.5 μg/mL. The cells on the glass coverslip were rinsed three times with PBS and placed upside-down on 3–5 μL of antifade mounting media (1 mg/mL p-phenylenediamine in 90% glycerol and 10% 20 mM Tris, pH 8.8) and sealed with nail polish. The labeled proteins were analyzed with a conventional fluorescent microscope equipped with a digital camera (Olympus, DP72) or a laser confocal microscope (Carl Zeiss LSM 700).
GST pull-down assay
HEK293 cells were seeded in 6 cm Petri dishes (1 × 106/Petri) and incubated for 20–24 h under the standard culture conditions. Then, the cells were co-transfected with pCHA-ACTB (3 µg) and pCAGGS-GST.cPA (2 µg) or pCAGGS-GST.ACTB (3 µg) and pCAGGS-cPA (2 µg) using PEI. After 48 h of transfection, the cells were rinsed with PBS and treated with 1 mM dithiobis (succinimidyl propionate (DSP) for cross-linking at 4°C for 60 min. The cells were then collected in 500 µL buffer A (150 mM NaCl, 50 mM Tris, pH 7.5, 1 mM EDTA, 0.1% NP-40, 1 mM PMSF) using a cell scraper and lysed with 10 cycles of freezing/thawing (liquid nitrogen/water bath at 37°C). After passing through a 26-gauge needle attached to a 1 mL syringe (10 times), the lysates were centrifuged at 15,000 rpm for 5 min at 4°C. The supernatants were used for protein precipitation with glutathione-Sepharose beads. For this, 200 μL of cell lysate, 300 μL of wash-binding buffer (150 mM NaCl, 50 mM Tris, 1 mM DTT, pH 8), and 50 μL of 50% slurry of glutathione-Sepharose beads were mixed and rotated with a vertical tube mixer for 4 h at 4°C. The glutathione-Sepharose beads were precipitated by centrifugation at 3,000 rpm for 2 min, and washed three times in 500 μL wash-binding buffer by rotating with a vertical tube mixer for 5 min. After the last wash, the precipitates were suspended in 50 μL of SDS-PAGE loading buffer, and the precipitated proteins were analyzed with SDS-PAGE/Western blotting.
Immunoprecipitation
As with the GST pull-down assay, the HEK293 cells were grown in 6 cm Petri dishes and co-transfected with plasmids coding viral cPA and HA-tagged β-actin (hACTB) proteins. After 48 h of transfection, the cells were cross-linked with DSP and harvested in 500 µL Buffer A as described above. 200 μL of cell lysate and 300 μL of wash-binding buffer (150 mM NaCl, 50 mM Tris, pH 8, 1 mM EDTA) were mixed in a 1.5 mL tube. After adding 4 μL of mouse monoclonal anti-HA antibody, the samples were mixed with a vertical mixer at 4°C for 2 h. Then, a 50 μL 50% slurry of protein A-Sepharose was added and mixed at 4°C overnight. After incubation, the protein A-Sepharose was washed three times with wash-binding buffer as described above and suspended in 50 µL of SDS-PAGE loading buffer. Precipitated proteins were analyzed with SDS-PAGE/Western blotting.
Over-expression and infection of the cells
The HEK293 cells were seeded in 12-well plates (2 × 105 cells/well) and transfected with 2 µg of plasmid DNA coding hACTB or deleted hACTB proteins. After 36 h of transfection, the medium was removed, and the cells were washed twice with OPTI-MEM (Gibco, United States). The cells were inoculated with A/WSN/33-H1N1 viruses diluted in 1% bovine serum albumin fraction V (BSA) at a 1–2 moi for 30 min. Then, the inoculum was removed, and the cells were incubated in a maintenance medium (DMEM containing 0.2% BSA fraction V and 4 μg/mL TPCK-trypsin) for 8 h. After incubation, the cells were lysed in a 200 µL SDS-PAGE loading buffer. Endogenous β-actin, viral proteins (PA and M1), and hACTB proteins encoded from the plasmids were analyzed with SDS-PAGE/Western blotting using mouse anti-actin, mouse anti-HA, rabbit anti-PA antibodies, and M1 rabbit antiserum.
Mini-replicon assay
The effects of over-expression of hACTB variants on the influenza A virus RdRp enzyme were investigated with the influenza A virus mini-replicon system as described above (Turan et al., 2004; Pham et al., 2018). The HEK293 cells were seeded in 24-well plates (5 × 104 cells/well) and co-transfected with a set of mini-replicon plasmids (pHH21-vNS-luc, pCAGGS-PB1, pCAGGS-PB2, pCAGGS-PA, and pCAGGS-NP) and 200 ng of a plasmid encoding the β-actin proteins. The plasmid DNA coding SEAP enzyme under the regulation of the Pol-II polymerase promoter was used as an internal control. After 48 h of transfection, the cells were lysed and luciferase activities in the lysates were defined with a commercial kit (Promega #E1483, Madison, United States) according to the manufacturer’s instructions.
Prediction of the protein interactions
In silico methods were used to predict possible interaction points between human β-actin and the influenza A virus PA protein. For this, homologous protein models were created. Based on the possible protein models, the interaction sites were predicted and analyzed by the protein-protein docking and the molecular visualization software. Three-dimensional structural models of the deleted β-actin and PA proteins were predicted by using the I-TASSER algorithm1 (Zhang, 2008). The best-predicted models were determined by using the C-score [−5 to +2], TM-score (>0.5), and root-mean-square deviation (RMSD). The putative complexes of β-actin and PA proteins were created using the ClusPro protein docking algorithm2 (Kozakov et al., 2017). In these complexes, the models with the highest number of cluster members and the lowest free energy were selected, and possible interaction sites and amino acids were determined with the PyMOL molecular visualization software.
Results
Identification of β-actin protein interaction with influenza a virus PA protein in yeast cells
Several host proteins that interact with influenza virus PA were identified using the Y2H screening assay carried out with the HEK293 Matchmaker cDNA library and the pGBD-cPA bait plasmid that expressed the GAL4-BD-cPA bait protein. The first step selection of candidate yeast colonies was carried out based on the histidine marker gene activated by protein-protein interaction which prevented the yeast growth in the medium lacking histidine in case of no interaction. The sequences of candidate cDNAs isolated from the yeast colonies grown on synthetic dextrose (SD) agar (lacking tryptophan, leucine, histidine and adenine), were identified with BLAST analysis. Among the identified genes, HCLS1 associated protein X-1 (HAX1), chromosome 14 open reading frame 166 (C14orf166), and minichromosome maintenance complex component 7 (MCM7) have been previously reported to be associated with influenza A viruses. In addition to these genes, the yeast cells carrying the cDNAs of human β-actin, mitochondrial ribosomal protein L27 (MRPL27), coiled-coil domain-containing protein 90B (CCD90B), small ubiquitin-like modifier 2 (SUMO2), fatty acid synthase (FASN), and ADP-ribosylation factor 4 (ARF4) were found to form colonies on SD media and to have high beta-galactosidase activity. In this study, considering the possible role of the above on the intracellular trafficking of influenza A virus vRNPs and/or virus transcription, we focused on the β-actin among them. Growing of the yeast cells expressing GAL4-BD-cPA bait and GAL4-AD-cDNA (β-actin) protein in SD medium without histidine showed that the viral PA and β-actin protein interact with each other in yeasts cells (Figure 1). The S. cerevisiae strain PJ69-4A carries the β-galactosidase reporter gene as a second marker that shows protein-protein interaction in the cells. The yeast cells expressing GAL4-BD-cPA bait protein and GAL4-AD-cDNA (β-actin) protein had a high β-galactosidase activity compared to the control cells (transformed with pGBD-cPA bait plasmid alone), supporting the interaction of viral PA and β-actin protein.
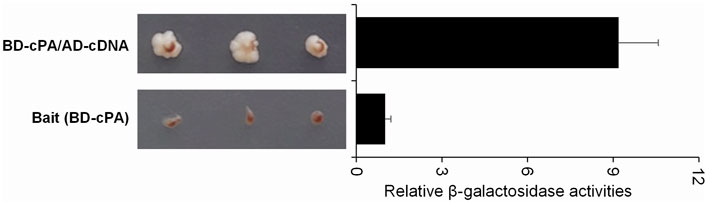
FIGURE 1. Growth profile of single- or double-transformed yeast cells on SD medium and relative β-galactosidase activities measured with o-Nitrophenyl-β-D-Galactopyranoside (ONPG) assays using saturated liquid yeast cultures. S. cerevisiae strain PJ69-4A yeast cells were transformed with the pGBD-cPA coding GAL4-BD-cPA bait protein (BD-cPA) (lower panel) or double transformed with the pGBD-cPA and pACT2-cDNA coding GAL4-activation domain fused with β-actin cDNA (AD-cDNA) (upper panel). To define the growth profiles, three colonies were cultured on SD agar plates (without Ade, His, Leu, and Trp). The β-galactosidase enzyme activities of the colonies were defined as described in the methods section.
β-actin protein interaction with the PA protein in mammalian cells
The relationship between the influenza A virus PA and β-actin was evaluated in HEK293 cells, the natural host cells for influenza A viruses with co-precipitation assays. In the first step, a GST pull-down assay was carried out, as mentioned above. The hACTB and cPA proteins, transiently expressed in the HEK293 cell, were co-precipitated with GST.cPA and GST.β-actin, respectively. The results indicated a direct interaction between β-actin and viral PA protein in mammalian cells (Figure 2A). This interaction of cPA and β-actin protein was also confirmed with a co-immunoprecipitation assay. Lysates were prepared from transfected HEK293 cells with pCHA-ACTB and pCAGGS-cPA or pCAGGS-nPA (Pham et al., 2018). Immunoprecipitation assays with anti-HA antibody against hACTB revealed that viral cPA was associated with β-actin in cells (Figure 2B). In contrast, the amino-terminal half of the PA (nPA) was not detected in the immunoprecipitates under the conditions employed here, indicating that the amino terminal half of the PA was not functional in this interaction (Figure 2B).
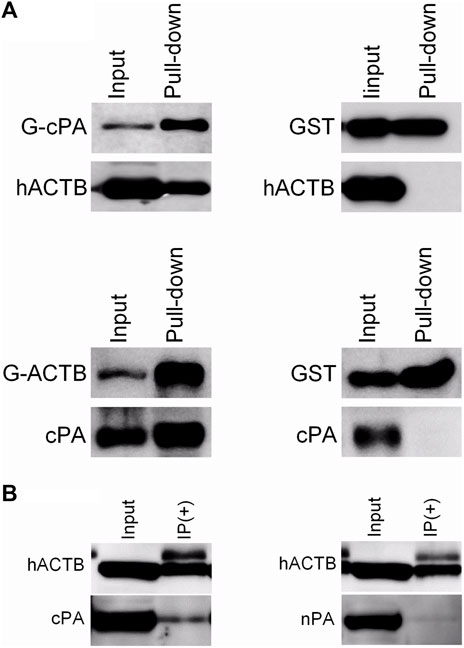
FIGURE 2. Co-precipitation results of the β-actin and the viral PA proteins. (A) GST pull-down with GST-cPA and GST-ACTB bait proteins. The proteins were transiently co-expressed in transfected HEK293 with pCHA-ACTB/pCAGGS-GST.cPA or pCAGGS-GST.ACTB/pCAGGS-cPA plasmids. GST-tagged proteins in the cell lysates were precipitated with glutathione-Sepharose beads. (B) Co-immunoprecipitation of the C-terminal (cPA) or N-terminal half (nPA) of viral PA proteins with hACTB. The hACTB proteins in lysates of transiently transfected HEK293 cell were precipitated with mouse monoclonal anti-HA antibody/protein A-Sepharose. The proteins in the precipitate were separated with SDS-PAGE, transferred to PVDF membrane and blotted with rabbit polyclonal anti-GST, rabbit polyclonal anti-PA or mouse monoclonal anti-HA antibodies, and then visualized using goat anti-rabbit or anti-mouse secondary antibodies conjugated to HRP and a chemiluminescence detection system.
The possible domains of the proteins involved in interaction of PA and β-actin were evaluated by immunoprecipitation assays by using hACTB variants, certain exon regions of which were removed by gene manipulations, and native PA, nPA and cPA proteins. The human β-actin gene is located on the short arm of chromosome 7 and codes a protein with ∼42 kD. This gene consists of six exons separated by introns. Considering the exon-intron junction points of the gene, four β-actin gene variants with deletions of certain exons were generated by inverse PCR from β-actin complete cDNA cloned into pCHA plasmid (pCHA-ACTB) (Figure 3A). The expressions of the HA-tagged β-actin (hACTB) and deleted β-actin proteins (hACTBΔ2, hACTBΔ2.3, hACTBΔ5.6, and hACTBΔ6) were detected with Western blotting in transiently transfected HEK293 cells (Figure 3B).
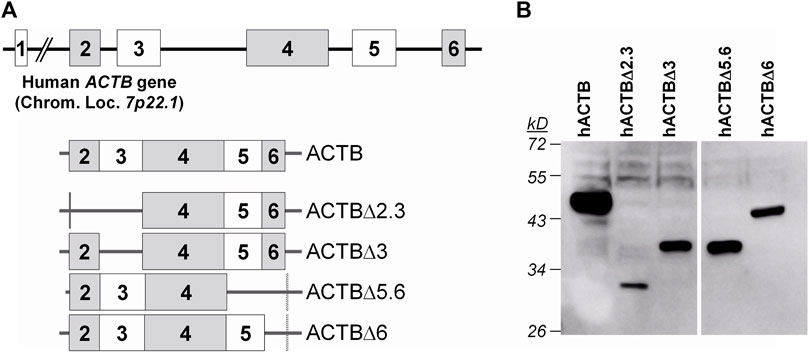
FIGURE 3. Human β-actin (ACTB) gene structure and native and deleted ACTB transcripts encoded from the plasmid vectors (A) and Western blot analysis of HA-tagged β-actin gene expressions in the transiently transfected HEK293 cells (B). The cells grown in a 12-well plate were transfected with 1 µg plasmid DNA, and, after 48 h of transfection, the cells were lysed in a 100 µL SDS-PAGE sample buffer. The proteins in the 10 µL sample were separated on 10% polyacrylamide gel and transferred to the PVDF membrane. The membrane was treated first with a mouse monoclonal anti-HA antibody, then a goat anti-mouse IgG conjugated to HRP, and visualized with a chemiluminescence detection system.
To analyze the interaction between viral PA and β-actin with the co-immunoprecipitation assay in detail, HEK293 cells were co-transfected with plasmid coding viral PA proteins (PA, nPA, or cPA) and β-actin variants. HA-tagged β-actin proteins co-expressed with viral PA proteins were precipitated with protein A-Sepharose by using a monoclonal anti-HA antibody, and both proteins in the precipitates were analyzed with Western blotting (Figure 4). It was observed that full-size PA protein co-precipitated with C-terminal deleted β-actins proteins (hACTBΔ5.6 and hACTBΔ6), but not with N-terminal deleted (hACTBΔ2.3) protein (Figure 4A). Furthermore, it was shown that the cPA protein co-precipitated in higher amounts with hACTBΔ5.6 and hACTBΔ6 proteins (Figure 4B). In contrast, the amino-terminal half of the PA (nPA) protein did not co-precipitated with the β-actin proteins (Figure 4C). This result showed that the amino-terminal domains of the β-actin protein bind to the carboxy-terminal moiety of the viral PA protein.
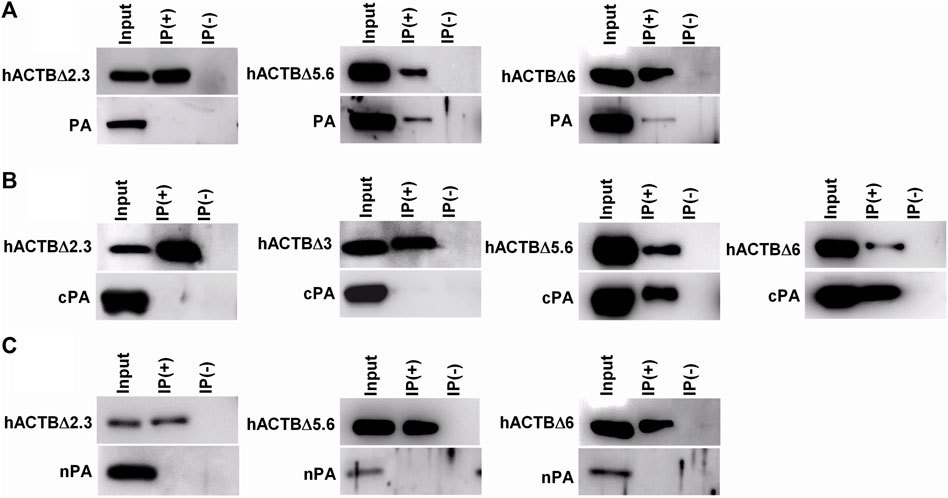
FIGURE 4. Analysis of β-actin and viral PA protein interaction with co-immunoprecipitation assay. The HEK293 cells grown in 6 cm petri dishes were co-transfected with the plasmid DNAs follows: (A) The plasmid coding influenza A virus PA (pCAGGS-PA) and a plasmid coding deleted β-actin (pCHA-hACTBΔ2.3, pCHA-hACTBΔ5.6 or pCHA-hACTBΔ6); (B) The plasmid DNA coding cPA (pCAGGS-PA) and a plasmid coding deleted β-actin (pCHA-hACTBΔ2.3, pCHA-hACTBΔ3, pCHA-hACTBΔ5.6, or pCHA-hACTBΔ6); (C) The plasmid DNA coding nPA (pCAGGS-nPA) and a plasmid coding deleted β-actin (pCHA-hACTBΔ2.3, pCHA-hACTBΔ5.6, or pCHA-hACTBΔ6). Forty-eight hours post-transfection, the cells were treated with DSP cross-linker and harvested in Buffer A. HA-tagged β-actin proteins in the cell lysates were precipitated with protein A-Sepharose using mouse monoclonal anti-HA antibodies. The proteins in precipitate were separated with SDS-PAGE, transferred to a PVDF membrane and blotted with rabbit polyclone anti-PA or mouse monoclonal anti-HA antibodies, and then visualized using goat anti-mouse or anti-rabbit secondary antibodies conjugated to HRP and a chemiluminescence detection system.
The intracellular localizations of β-actin and viral PA support of the interaction of these proteins in mammalian cells
Intracellular localization of transiently expressed β-actin variants and/or viral PA proteins in HeLa cells were determined with an immunofluorescence assay by using mouse monoclonal anti-HA and rabbit polyclonal anti-PA antibodies, respectively. The endogenous filamentous actin proteins were labelled with Alexa 568-Phalloidin. It was determined that the hACTB protein has a radial localization in the cell cytoplasm, similar to endogenous filamentous actin, mostly in regions close to the cell membrane. hACTBΔ2 and hACTBΔ2.3, amino-terminal deleted variants, localize as aggregates homogeneously distributed in the cell cytoplasm, incompatible with the hACTB. Carboxy-terminal deleted hACTBΔ5.6 and hACTBΔ6 showed the cytoplasm localization similar to the hACTB, close to the cell membrane (Figure 5A). Similar localization patterns of HA-tagged β-actin variants are also observed in the HeLa cells whose the filamentous actin proteins are labeled with Alexa 568-phalloidin (Figure 5B).
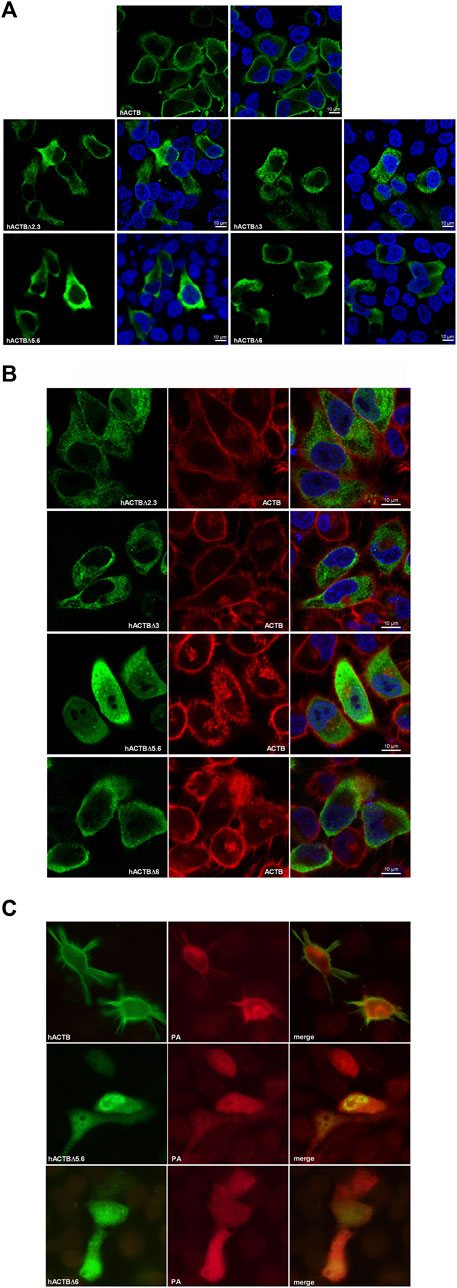
FIGURE 5. Intracellular localization of HA-tagged β-actin, viral PA proteins and endogenous filamentous actin in the HeLa cells transfected with the plasmid vectors. (A) Localization of β-actin proteins in HeLa cells transfected with a β-actin expression plasmid alone. The proteins were labeled with monoclonal mouse anti-HA primary antibody and Alexa-488 conjugated anti-mouse IgG secondary antibody. (B) The filamentous actin proteins labeled with Alexa 568-Phalloidin in the cells transiently expressing the β-actin variants. (C) The intracellular localization of viral PA and β-actin proteins co-expressed in the HeLa cells transfected with the plasmids DNAs. The proteins were labeled with polyclonal rabbit anti-PA/monoclonal mouse anti-HA primary antibodies and Alexa-568 conjugated anti-rabbit IgG/Alexa-488 conjugated anti-mouse IgG antibodies. The cells were observed by using a laser confocal microscope (Carl Zeiss LSM 700) and a conventional fluorescence microscope (Olympus BX50).
The influenza A virus PA protein encoded by the plasmid DNA shows nucleo-cytoplasmic localization in the HeLa cells. The carboxy-terminal domain deleted β-actins (ACTBΔ5.6 and ACTBΔ6), having a similar localization pattern with hACTB when expressed alone, also show nucleo-cytoplasmic localization with the viral PA protein in the cells (Figure 5C). This co-localization patterns supported the interaction of amino terminal domains of β-actin with viral PA protein.
No significant effect on the influenza a virus replication of overexpression of deleted β-actin variants
During viral infection, there is a complex and tight interaction between the host cell and viral proteins which may affect viral replication. To detect the possible effects of the β-actin protein on the viral replication, the HEK293 cells over-expressing the HA-tagged ACTB variants were infected with influenza A WSN viruses, and 8 h after infection, the viral proteins and both endogenous β-actin (ACTB) and transiently expressed β-actin proteins (hACTBs) were analyzed with SDS-PAGE/Western blotting (Figure 6A). The results showed that over-expression of the hACTB had a low negative effect on the viral protein level. In contrast, deleted hACTB proteins had no significant effect on the viral replication at the early stage of infection. The effects of β-actin variants on virus RNA polymerase were also investigated in transiently transfected HEK293 cells by using the influenza A virus mini-genome. The luciferase enzyme activities, which are dependent on the viral polymerase enzyme in the cell lysates, were determined. The results showed that the over-expression of deleted hACTB proteins created no significant change in reported luciferase activities. In contrast, the HA-tagged native β-actin (hACTB) protein resulted in an average 65% decrease of reporter luciferase activity in the cells compared to the control (Figure 6B).
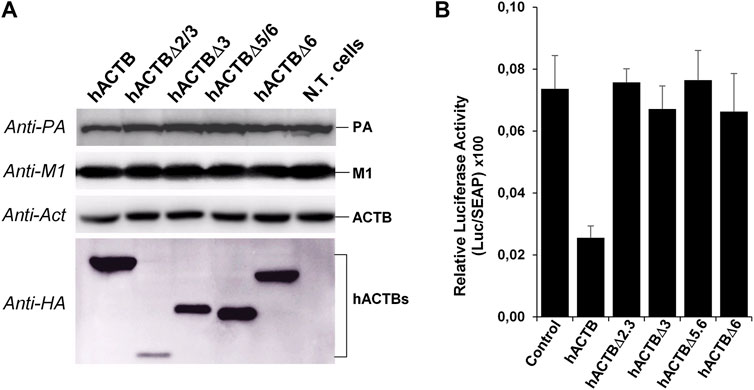
FIGURE 6. Effects of overexpression of β-actin proteins on influenza A virus replication (A) and viral RdRp activity (B) in transfected HEK293 cells. (A) The cells grown in a 12-well plate were transfected with 2 µg plasmid DNA. After 36 h of transfection, the cells were infected with influenza A viruses at a multiplicity of infection (MOI) of 1 for 8 h. The viral proteins (PA and M1), endogenous β-actin (ACTB), and HA-tagged β-actin proteins were analyzed with Western blotting. (B) The HEK293 cells were grown in a 24-well plate and co-transfected with a 200 ng of β-actin expression plasmid and influenza A virus mini-replicon plasmids (for each well: 10 ng of pHH21-vNS-luc, 10 ng of pCAGGS-PB2, 10 ng of pCAGGS-PB1, 3 ng of pCAGGS-PA, and 50 ng of pCAGGS-NP; 20 ng of pSEAP control plasmid), and the reporter enzyme activities were defined as previously described.
In silico prediction of human ACTB and influenza A PA interaction
The co-precipitation of β-actin protein with viral PA protein in GST pull down and immunoprecipitation assays is an important indicator that these two proteins interact with each other in mammalian cells as well. Although truncated proteins are not native proteins, the co-precipitation of C-terminal deleted β-actin variants with the viral PA protein suggests that the N-terminal region of the protein may be important in this interaction. The fact that many factors interfere with protein interactions in the cell makes it extremely difficult to reveal binding patterns. Here, possible interaction patterns were predicted by in silico algorithms, ignoring the intracellular factors and infection conditions. In these interaction patterns, the models with the highest values of cluster number and the lowest free energy were selected, and the possible interaction sites and amino acid residues were determined with the PyMOL molecular visualization software. Considering the co-precipitation properties of the deleted proteins and docking assessment results, the first and most probable interaction patterns for the protein complexes are given in Figure 7. In these models, the amino acid residues in N-terminal halves of β-actin, hACTBΔ5.6 and hACTBΔ6 proteins seem to be prominent. These patterns agree with the results of the co-immunoprecipitation results.
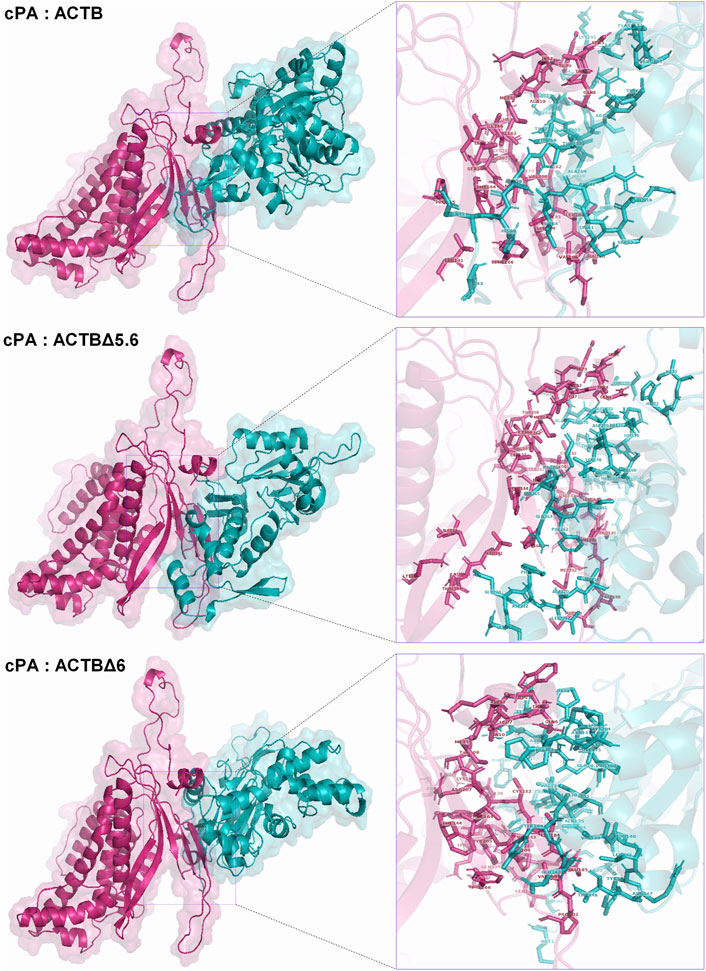
FIGURE 7. In silico prediction of interactions between influenza cPA and human β-actin proteins. Three-dimensional structural and putative complexes of β-actin and PA proteins were generated by I-TASSER and ClusPro protein docking algorithms, respectively. Possible interaction sites and amino acids were determined with the PyMOL molecular visualization software (right panel).
Discussion
Influenza virus RdRp subunits are not only involved in transcription and replication of viral genome but also play a crucial role in overcoming the host species barrier by interacting with several host cellular proteins (Naffakh et al., 2008; Manz et al., 2013). Here we considered the interaction of the host β-actin protein, which was determined by the Y2H method as a candidate interactor, with the PA subunit of the viral RdRp in mammalian cells. The β-actin proteins are assembled as actin filaments (Dominguez and Holmes, 2011) form the cytoskeleton, together with microtubules and intermediate filaments and give a shape to the cells. It has been shown that the actin microfilaments also function in the intracellular replication of many viruses, the transport and establishment of viral components, and the release of the virion by budding. The vaccinia virus, having a DNA genome, benefits from the cytoskeleton formed by actin and tubulins to be localized in the viral genome in the right place at the right time and to perform an effective infection in the host cell (Krauss et al., 2002; Smith et al., 2002). Polioviruses with a positive-sense RNA genome utilize the actin cytoskeleton during infection; after attaching to the cell, the virion is engulfed by an actin-dependent pathway followed by the release of the RNA genome (Brandenburg et al., 2007). Not only naked viruses such as poliovirus but also enveloped viruses such as the herpes simplex virus (Feierbach et al., 2006), respiratory syncytial viruses (Shahriari et al., 2016; Shahriari et al., 2018), and the Sendai virus (Chandra et al., 2017) interact with the cellular actins (Taylor et al., 2011). These data show that the proteins constituting the cytoskeleton are essential for the replication of many viruses. In this study, we showed that the carboxy-terminal moiety of the PA protein (cPA), which is used as a bait in Y2H screening assay, has positive interaction with the human β-actin protein (Figure 1). This result also indicates that the carboxy-terminal moiety of the PA protein is involved in the interaction with β-actin. The interaction of viral PA protein with β-actin in mammalian cells was also investigated. Both the GST pull down and co-immunoprecipitation assays showed that the PA protein co-precipitates with β-actin proteins in transiently transfected cell lysates (Figure 2). Avalos et al. (1997) reported that the influenza virus NP and M1 proteins interact with cellular microfilaments. The viral PA protein as a subunit of the viral RdRp enzyme is carried out together with viral RNA molecules which are complexed with NP proteins as a vRNP (Klumpp et al., 1997). It is possible that the PA protein, which is a part of the vRNP complex, may have a role in the intracellular trafficking of the vRNPs in contact with actin filaments. It is also possible that the β-actin protein interferes with influenza virus transcription via the PA protein. Although actin protein is mostly found in the cell cytoplasm, it has been determined that approximately 25% of actin are located in the nucleus (Dopie et al., 2012). The actin protein is not only involved in cell movement and the positioning of intracellular organelles (Pollard and Cooper, 2009), but also have a role in the regulation of gene expression (Zheng et al., 2009). Therefore, the possible role of actin in influenza viral transcription, which take place in the cell nucleus because of the dependence of viral RdRp to the 5′-cap structure of the nascent mRNA, should not be overlooked (Dias et al., 2009; De Vlugt et al., 2018). The over-expression of the ACTB protein caused a very limited decrease in the viral protein level in the early stage of infection compared to the control (Figure 6A). It was concluded that this was due to the excess amount of endogenous β-actin in the cells. However, the over-expression of the hACTB protein significantly inhibited the influenza A virus RdRp in the mini-replicon assay (Figure 6B). This inhibition may be the result of the indirect effect of β-actin protein which is known to affect the transcription of some genes or may result in an effect on the viral RdRp complex via the PA subunit.
In order to obtain clues regarding which domains might be important in binding to the viral PA protein, the domains in the amino- and carboxy-terminal regions of β-actin protein corresponding to the exons 2/3 and exons 5/6 of the gene were deleted. The interaction of the deleted β-actin variants with the PA proteins was evaluated by co-immunoprecipitation. The results showed that the amino-terminal region of the β-actin protein is important in interacting with the viral cPA protein (Figure 4). The localization patterns of the β-actin and PA proteins when co-expressed or individually synthesized in the transfected cells, which was revealed with immunofluorescence assays, also support the interaction of these two proteins (Figures 5A, C). The intracellular distribution of proteins truncated at the amino-terminal regions (hACTBΔ2 and hACTBΔ2.3) in transfected cells such as homogeneous aggregates suggests that these proteins do not participate in the filamentous actin structures (Figure 5B). Although the localization patterns of carboxy-terminal deleted ACTB proteins (hACTBΔ6 and hACTBΔ5.6) are similar to filamentous actin, it is somewhat difficult to say that they participate in the filamentous actin structure.
The in silico algorithms developed for the prediction of three-dimensional (3D) structure and interaction patterns of the proteins make an important contribution to our understanding of the relationship and function of the proteins in the cells. However, the complexity of protein interactions within the cell, the involvement of environmental conditions, and various factors such as chaperones in the formation of protein 3D structure make in silico predictions very difficult. In this study, considering the experimental data obtained, possible interaction patterns between hACTB, hACTBΔ6, hACTBΔ5.6 (omitted HA tag in prediction), and viral cPA proteins were defined with ClusPro algorithms (Figure 7). In silico predicted 3D structures and docking patterns suggested that the amino-terminal part of actin and the carboxy-terminal region of the viral PA protein are important in this interaction.
In conclusion, the human β-actin protein was defined as a new interactor host protein factor of influenza A virus RdRp by using a Y2H assay. This interaction was confirmed in mammalian cells. It was demonstrated that the carboxy-terminal half of the PA and the amino-terminal region of the β-actin protein were involved in this interaction. It was difficult to draw a conclusion about the importance of this association for influenza A virus replication from these data. However, it has been suggested that actin proteins may have a role in the intracellular trafficking of vRNPs via the PA subunit of the RdRp enzyme, which is a component of vRNPs, or interfere in the viral RNA transcription.
Data availability statement
The original contributions presented in the study are included in the article/supplementary material, further inquiries can be directed to the corresponding author.
Author contributions
NG: conducted experiments. EÇ: performed data analysis, contributed to wrote and drafted the manuscript, critical revision of the article. KT: research conception and design, conducted experiments, wrote and drafted the manuscript. All authors contributed to the article and approved the submitted version.
Funding
This work was supported by a grant from the Scientific and Technological Research Council of Turkey (TÜBİTAK, grant no. SBAG-112S518).
Conflict of interest
The authors declare that the research was conducted in the absence of any commercial or financial relationships that could be construed as a potential conflict of interest.
Footnotes
1https://zhanglab.ccmb.med.umich.edu/I-TASSER/
References
Avalos, B. T., Yu, Z., and Nayak, D. P. (1997). Association of influenza virus NP and M1 proteins with cellular cytoskeletal elements in influenza virus infected cells. J. Virol. 71, 2947–2958. doi:10.1128/jvi.71.4.2947-2958.1997
Brandenburg, B., Lee, L. Y., Lakadamyali, M., Rust, M. J., Zhuang, X., and Hogle, J. M. (2007). Imaging poliovirus entry in live cells. PLoS Biol. 5 (7), e183. doi:10.1371/journal.pbio.0050183
Bunnell, T. M., Burbach, B. J., Shimizu, Y., and Ervasti, J. M. (2011). β-Actin specifically controls cell growth, migration, and the G-actin pool. Mol. Biol. Cell 22 (21), 4047–4058. doi:10.1091/mbc.e11-06-0582
Chandra, S., Kalaivani, R., Kumar, M., Srinivasan, N., and Sarkar, D. P. (2017). Sendai virus recruits cellular villin to remodel actin cytoskeleton during fusion with hepatocytes. Mol. Biol. Cell 28 (26), 3801–3814. doi:10.1091/mbc.e17-06-0400
Couch, R. B. (1996). “Orthomyxoviruses,” in Medical microbiology. Editor S. Baron (Galveston (TX): University of Texas Medical Branch at Galveston).
De Vlugt, C., Sikora, D., and Pelchat, M. (2018). Insight into influenza: a virus cap-snatching. Viruses 10 (11), 641. doi:10.3390/v10110641
Dias, A., Bouvier, D., Crepin, T., McCarthy, A. A., Hart, D. J., Baudin, F., et al. (2009). The cap-snatching endonuclease of influenza virus polymerase resides in the PA subunit. Nature 458 (7240), 914–918. doi:10.1038/nature07745
Dominguez, R., and Holmes, K. C. (2011). Actin structure and function. Annu. Rev. Biophys. 40, 169–186. doi:10.1146/annurev-biophys-042910-155359
Dopie, J., Skarp, K. P., Rajakyla, E. K., Tanhuanpaa, K., and Vartiainen, M. K. (2012). Active maintenance of nuclear actin by importin 9 supports transcription. Proc. Natl. Acad. Sci. U. S. A. 109 (9), E544–E552. doi:10.1073/pnas.1118880109
Dou, D., Revol, R., Ostbye, H., Wang, H., and Daniels, R. (2018). Influenza A virus cell entry, replication, virion assembly and movement. Front. Immunol. 9, 1581. doi:10.3389/fimmu.2018.01581
Feierbach, B., Piccinotti, S., Bisher, M., Denk, W., and Enquist, L. W. (2006). Alpha-herpesvirus infection induces the formation of nuclear actin filaments. PLoS Pathog. 2 (8), e85. doi:10.1371/journal.ppat.0020085
Gao, Z., Hu, J., Wang, X., Yang, Q., Liang, Y., Ma, C., et al. (2018). The PA-interacting host protein nucleolin acts as an antiviral factor during highly pathogenic H5N1 avian influenza virus infection. Arch. Virol. 163 (10), 2775–2786. doi:10.1007/s00705-018-3926-3
Gietz, R. D., and Schiestl, R. H. (2007). Large-scale high-efficiency yeast transformation using the LiAc/SS carrier DNA/PEG method. Nat. Protoc. 2 (1), 38–41. doi:10.1038/nprot.2007.15
He, F., Ling, L., Liao, Y., Li, S., Han, W., Zhao, B., et al. (2014). Beta-actin interacts with the E2 protein and is involved in the early replication of classical swine fever virus. Virus Res. 179, 161–168. doi:10.1016/j.virusres.2013.10.016
Hoang, H. D., Neault, S., Pelin, A., and Alain, T. (2021). Emerging translation strategies during virus-host interaction. Wiley Interdiscip. Rev. RNA 12 (1), e1619. doi:10.1002/wrna.1619
Hsu, W. B., Shih, J. L., Shih, J. R., Du, J. L., Teng, S. C., Huang, L. M., et al. (2013). Cellular protein HAX1 interacts with the influenza A virus PA polymerase subunit and impedes its nuclear translocation. J. Virol. 87 (1), 110–123. doi:10.1128/jvi.00939-12
James, P., Halladay, J., and Craig, E. A. (1996). Genomic libraries and a host strain designed for highly efficient two-hybrid selection in yeast. Genetics 144 (4), 1425–1436. doi:10.1093/genetics/144.4.1425
Kawaguchi, A., Naito, T., and Nagata, K. (2005). Involvement of influenza virus PA subunit in assembly of functional RNA polymerase complexes. J. Virol. 79 (2), 732–744. doi:10.1128/jvi.79.2.732-744.2005
Klumpp, K., Ruigrok, R. W., and Baudin, F. (1997). Roles of the influenza virus polymerase and nucleoprotein in forming a functional RNP structure. EMBO J. 16 (6), 1248–1257. doi:10.1093/emboj/16.6.1248
Kozakov, D., Hall, D. R., Xia, B., Porter, K. A., Padhorny, D., Yueh, C., et al. (2017). The ClusPro web server for protein-protein docking. Nat. Protoc. 12 (2), 255–278. doi:10.1038/nprot.2016.169
Krauss, O., Hollinshead, R., Hollinshead, M., and Smith, G. L. (2002). An investigation of incorporation of cellular antigens into vaccinia virus particles. J. Gen. Virol. 83 (Pt 10), 2347–2359. doi:10.1099/0022-1317-83-10-2347
Longo, P. A., Kavran, J. M., Kim, M. S., and Leahy, D. J. (2013). Transient mammalian cell transfection with polyethylenimine (PEI). Methods Enzymol. 529, 227–240. doi:10.1016/B978-0-12-418687-3.00018-5
Lui, Y. L., Lin, Z., Lee, J. J., Chow, V. T., Poh, C. L., and Tan, E. L. (2013). Beta-actin variant is necessary for Enterovirus 71 replication. Biochem. Biophys. Res. Commun. 433 (4), 607–610. doi:10.1016/j.bbrc.2013.03.044
Ma, C., Li, Y., Zong, Y., Velkov, T., Wang, C., Yang, X., et al. (2022). p21 restricts influenza A virus by perturbing the viral polymerase complex and upregulating type I interferon signaling. PLoS Pathog. 18 (2), e1010295. doi:10.1371/journal.ppat.1010295
Manz, B., Schwemmle, M., and Brunotte, L. (2013). Adaptation of avian influenza A virus polymerase in mammals to overcome the host species barrier. J. Virol. 87 (13), 7200–7209. doi:10.1128/jvi.00980-13
Mehle, A., Dugan, V. G., Taubenberger, J. K., and Doudna, J. A. (2012). Reassortment and mutation of the avian influenza virus polymerase PA subunit overcome species barriers. J. Virol. 86 (3), 1750–1757. doi:10.1128/jvi.06203-11
Naffakh, N., Tomoiu, A., Rameix-Welti, M. A., and van der Werf, S. (2008). Host restriction of avian influenza viruses at the level of the ribonucleoproteins. Annu. Rev. Microbiol. 62, 403–424. doi:10.1146/annurev.micro.62.081307.162746
Nagata, K., Saito, S., Okuwaki, M., Kawase, H., Furuya, A., Kusano, A., et al. (1998). Cellular localization and expression of template-activating factor I in different cell types. Exp. Cell Res. 240 (2), 274–281. doi:10.1006/excr.1997.3930
Pflug, A., Lukarska, M., Resa-Infante, P., Reich, S., and Cusack, S. (2017). Structural insights into RNA synthesis by the influenza virus transcription-replication machine. Virus Res. 234, 103–117. doi:10.1016/j.virusres.2017.01.013
Pham, P. T. V., Turan, K., Nagata, K., and Kawaguchi, A. (2018). Biochemical characterization of avian influenza viral polymerase containing PA or PB2 subunit from human influenza A virus. Microbes Infect. 20 (6), 353–359. doi:10.1016/j.micinf.2018.04.003
Pirincal, A., and Turan, K. (2021). Human DDX56 protein interacts with influenza A virus NS1 protein and stimulates the virus replication. Genet. Mol. Biol. 44 (1), e20200158. doi:10.1590/1678-4685-gmb-2020-0158
Pollard, T. D., and Cooper, J. A. (2009). Actin, a central player in cell shape and movement. Science 326 (5957), 1208–1212. doi:10.1126/science.1175862
Senbas-Akyazi, B., Pirincal, A., Kawaguchi, A., Nagata, K., and Turan, K. (2020). Interaction of influenza A virus NS2/NEP protein with the amino-terminal part of Nup214. Turk J. Biol. 44 (2), 82–92. doi:10.3906/biy-1909-49
Shahriari, S., Gordon, J., and Ghildyal, R. (2016). Host cytoskeleton in respiratory syncytial virus assembly and budding. Virol. J. 13 (1), 161. doi:10.1186/s12985-016-0618-z
Shahriari, S., Wei, K. J., and Ghildyal, R. (2018). Respiratory syncytial virus matrix (M) protein interacts with actin in vitro and in cell culture. Viruses 10 (10), 535. doi:10.3390/v10100535
Smith, G. L., Vanderplasschen, A., and Law, M. (2002). The formation and function of extracellular enveloped vaccinia virus. J. Gen. Virol. 83 (Pt 12), 2915–2931. doi:10.1099/0022-1317-83-12-2915
Stuven, T., Hartmann, E., and Gorlich, D. (2003). Exportin 6: A novel nuclear export receptor that is specific for profilin.actin complexes. EMBO J. 22 (21), 5928–5940. doi:10.1093/emboj/cdg565
Sugiyama, K., Kawaguchi, A., Okuwaki, M., and Nagata, K. (2015). pp32 and APRIL are host cell-derived regulators of influenza virus RNA synthesis from cRNA. Elife 4, e08939. doi:10.7554/elife.08939
Sun, Y., Xu, Q., Shen, Y., Liu, L., Wei, K., Sun, H., et al. (2014). Naturally occurring mutations in the PA gene are key contributors to increased virulence of pandemic H1N1/09 influenza virus in mice. J. Virol. 88 (8), 4600–4604. doi:10.1128/jvi.03158-13
Takizawa, N., Watanabe, K., Nouno, K., Kobayashi, N., and Nagata, K. (2006). Association of functional influenza viral proteins and RNAs with nuclear chromatin and sub-chromatin structure. Microbes Infect. 8 (3), 823–833. doi:10.1016/j.micinf.2005.10.005
Taylor, M. P., Koyuncu, O. O., and Enquist, L. W. (2011). Subversion of the actin cytoskeleton during viral infection. Nat. Rev. Microbiol. 9 (6), 427–439. doi:10.1038/nrmicro2574
Te Velthuis, A. J., and Fodor, E. (2016). Influenza virus RNA polymerase: insights into the mechanisms of viral RNA synthesis. Nat. Rev. Microbiol. 14 (8), 479–493. doi:10.1038/nrmicro.2016.87
Turan, K., Mibayashi, M., Sugiyama, K., Saito, S., Numajiri, A., and Nagata, K. (2004). Nuclear MxA proteins form a complex with influenza virus NP and inhibit the transcription of the engineered influenza virus genome. Nucleic Acids Res. 32 (2), 643–652. doi:10.1093/nar/gkh192
Turan, K., Nagata, K., and Kuru, A. (1996). Antiviral effect of Sanicula europaea L. leaves extract on influenza virus-infected cells. Biochem. Biophys. Res. Commun. 225 (1), 22–26. doi:10.1006/bbrc.1996.1125
Wang, J., Fang, S., Xiao, H., Chen, B., Tam, J. P., and Liu, D. X. (2009). Interaction of the coronavirus infectious bronchitis virus membrane protein with beta-actin and its implication in virion assembly and budding. PLoS One 4 (3), e4908. doi:10.1371/journal.pone.0004908
York, A., and Fodor, E. (2013). Biogenesis, assembly, and export of viral messenger ribonucleoproteins in the influenza A virus infected cell. RNA Biol. 10 (8), 1274–1282. doi:10.4161/rna.25356
Zhang, Y. (2008). I-TASSER server for protein 3D structure prediction. BMC Bioinforma. 9, 40. doi:10.1186/1471-2105-9-40
Keywords: influenza A viruses, influenza RdRp, PA protein, β-actin, yeast two-hybrid
Citation: Gelmez N, Çağlayan E and Turan K (2024) The interaction of influenza A virus RNA polymerase PA subunit with the human β-actin protein. Acta Virol. 67:11890. doi: 10.3389/av.2023.11890
Received: 08 July 2022; Accepted: 07 July 2023;
Published: 08 January 2024.
Edited by:
Katarina Polcicova, Slovak Academy of Sciences, SlovakiaCopyright © 2024 Gelmez, Çağlayan and Turan. This is an open-access article distributed under the terms of the Creative Commons Attribution License (CC BY). The use, distribution or reproduction in other forums is permitted, provided the original author(s) and the copyright owner(s) are credited and that the original publication in this journal is cited, in accordance with accepted academic practice. No use, distribution or reproduction is permitted which does not comply with these terms.
*Correspondence: Kadir Turan, a2FkaXJ0dXJhbkBtYXJtYXJhLmVkdS50cg==
†ORCID: Nazife Gelmez, orcid.org/0000-0002-4908-743X; Elif Çağlayan, orcid.org/0000-0002-4249-0824; Kadir Turan, orcid.org/0000-0003-4175-3423