- 1Institute of Virology, Biomedical Research Center of the Slovak Academy of Sciences, Bratislava, Slovakia
- 2Faculty of Natural Sciences, University of Ss. Cyril and Methodius, Trnava, Slovakia
Grapevine yellow speckle viroid 1 (GYSVd-1) is a widespread subviral pathogen affecting grapevine (Vitis vinifera L.), with potential implications for viticulture. This study investigates the prevalence, genetic variability, intrahost population polymorphism and secondary RNA structure of GYSVd-1 in Slovakia, where its molecular characteristics have been largely unexplored. Leaf samples from 49 grapevine plants across western and southern Slovakia were analyzed using RT-PCR, followed by sequencing and phylogenetic analyses. GYSVd-1 was detected in 73% of the samples, demonstrating high prevalence rates across vineyards in Slovakia. Sequence analysis revealed a high degree of genetic similarity among Slovak isolates, with nucleotide identity ranging from 96.2% to 100%, consistent with low variability reported globally. Phylogenetic analyses showed no specific grouping of Slovak isolates. Intrahost variability, assessed via subcloning of RT-PCR products, demonstrated the presence of random mutations among viroid clones, consistent with quasispecies nature of viroids in their hosts. These findings suggest that both interhost and intrahost viroid populations evolve under similar pressures to maintain structural stability essential for viroid function. Secondary RNA structure analysis of Slovak GYSVd-1 isolates revealed that all unambiguous sequences exhibited the characteristic rod-like conformation. In contrast, a significant proportion of sequences from mixed populations failed to form proper rod-like structures, suggesting that these variants may lack biological viability. Overall, this study unveils the prevalence of GYSVd-1 in Slovak vineyards and provides valuable insights to guide future phylogenetic studies and structural analyses of viroids.
Introduction
Grapevine yellow speckle viroid 1 (Apscaviroid alphaflavivitis, GYSVd-1) is one of the most widespread viroids infecting grapevine (Vitis vinifera L.), belonging to the Pospiviroidae family (genus Apscaviroid).1 Viroids are small, circular single-stranded RNA molecules, lacking a protein-coding capacity yet capable of hijacking host cellular machinery to replicate and cause disease (Diener, 2001). First described in association with grapevine diseases characterized by yellow speckling on leaves (Taylor and Woodham, 1972; Koltunow and Rezaian, 1988; Koltunow et al., 1989), GYSVd-1 has since been detected in various grapevine-growing regions worldwide (Ward et al., 2011; Sahana et al., 2013; Gambino et al., 2014; Vozárová et al., 2016; Fajardo et al., 2016; Jo et al., 2017; Zongoma et al., 2018; Delić et al., 2018). The economic significance of GYSVd-1 lies in its potential to compromise the health and productivity of grapevines, a crop of immense value to the global wine and fruit industries (Hadidi et al., 2017). Although GYSVd-1 infections often remain asymptomatic under certain weather conditions (Hadidi et al., 2017), its presence in grapevines can contribute to complex interactions with other pathogens, worsening symptoms and reducing fruit quality and vine vigour (Martelli, 2014; Abdullah et al., 2017). Moreover, its efficient transmissibility through vegetative propagation underscores its potential to spread within and between vineyards, posing a significant challenge for disease management (Habili, 2017). Despite its ubiquity, the epidemiology and molecular diversity of GYSVd-1 remain understudied in several grapevine-growing regions, including Slovakia. Following a preliminary study, GYSVd-1 was first reported in Slovakia in 2016 (Vozárová et al., 2016). However, its prevalence and molecular characteristics in Slovak vineyards have remained largely unexplored until now. Molecular characterization of GYSVd-1 is essential to understand its genetic variability, which can influence its pathogenicity, transmission efficiency, and adaptation to environmental conditions. Previous studies from different regions have shown low sequence and structural heterogeneity among GYSVd-1 isolates (Rigden and Rezaian, 1993; Szychowski et al., 1998; Sahana et al., 2013; Fajardo et al., 2016). However, there is still a lack of data on GYSVd-1 prevalence and genetic diversity in Central European vineyards, leaving a gap in our understanding of its regional dynamics. This study investigates the presence, prevalence, and molecular variability of GYSVd-1 isolates infecting grapevines in Slovakia, where viticulture is a vital agricultural sector. Understanding the distribution and variability of GYSVd-1 will contribute to the development of effective management strategies to mitigate its impact on viticulture.
Materials and methods
Sample collection and total RNA extraction
Leaf samples (n = 49) were collected from different cultivars of grapevine (Vitis vinifera L.) in vineyards across western and southern Slovakia during the growing seasons of 2023 and 2024 (May-October). None of the sampled grapevines exhibited symptoms typically associated with Grapevine Yellow Speckle Disease, although various non-specific virus-like or physiological disorders were observed. Plants were therefore selected randomly and independently of visible symptomatology to ensure a representative sampling of the grapevine population, including asymptomatic carriers. Fully developed leaves were collected from different parts of each plant and stored on ice for transportation to the laboratory, where they were processed for nucleic acid extraction. Total RNA was extracted from the midrib of collected grapevine leaves (approx. 100 mg, 3 leaves per plant) using the Spectrum™ Plant Total RNA Kit (Sigma-Aldrich, St. Louis, MO, United States), following the manufacturer’s protocol. RNA concentrations and purity were assessed spectrophotometrically (Nanodrop 2000c, Thermo Fisher Scientific, Waltham, MA, United States) and the samples were stored at −80°C until further analysis.
Reverse transcription polymerase chain reaction (RT-PCR)
Total RNA extracted from the grapevine samples was used as a template for two-step reverse transcription PCR (RT-PCR). First-strand cDNA synthesis was performed using AMV Reverse Transcriptase and random primers (Promega, Madison, WI, United States), following the manufacturer’s instructions. The synthesized cDNA was subsequently used as a template for PCR amplification. Amplification was carried out using proofreading TaKaRa Ex Taq polymerase (Takara Bio Inc., Shiga, Japan) and a GYSVd-specific primer pair 5′-TAAGAGGTCTCCGGATCTTCTTGC-3´ (sense) and 5′-GCGGGGGTTCCGGGGATTGC-3´ (antisense), enabling to obtain complete viroid sequence as described by Elleuch et al. (2013). These primers are designed to anneal within the left terminal domain of the GYSVd-1 genome. Their binding sites are positioned directly adjacent to each other (Figure 1), enabling the amplification of the entire circular GYSVd-1 genome. PCR was conducted under the following cycling conditions: initial denaturation at 95°C for 3 min; 35 cycles of denaturation at 95°C for 15 s, annealing at 60°C for 30 s, and elongation at 72°C for 25 s; followed by a final elongation step at 72°C for 5 min. Amplified products were analyzed by electrophoresis in 3% agarose gel (w/v). Selected amplicons were purified from the reaction mixture using the Wizard® SV Gel and PCR Clean-Up System kit (Promega, Madison, WI, United States), according to the manufacturer’s protocol.
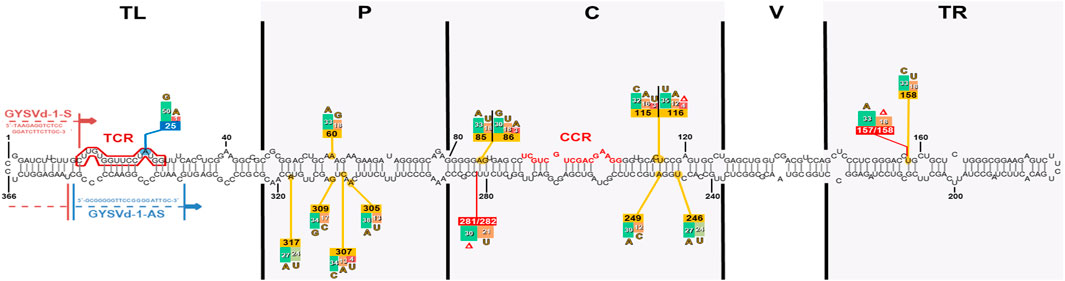
Figure 1. Mutational hotspots based on the alignment of all Slovak GYSVd-1 isolates mapped on the secondary RNA structure of isolate RV140 clone 1 (Gibbs free energy dG = −152,10 kcal/mol). This isolate, representing a shorter sequence variant (366 nts), was used as a template to display all mutations identified across Slovak GYSVd-1 isolates and clones, exhibiting both rod-like and non-rod-like structures. Positions with the highest frequency of substitutions (yellow boxes) and deletions (red boxes) are highlighted, predominantly in the pathogenicity and central domains. Numbers within the boxes indicate the frequency of mutations across all 51 analyzed sequences, reflecting the most variable regions of the GYSVd-1 genome. Other observed mutated positions are not shown due to their low frequency (for example position 25 – blue box). Binding sites of the primers used in this study (Elleuch et al., 2013) are also indicated. TL, terminal left domain; P, pathogenicity domain; C, central conserved domain; V, variable domain; TR, terminal right domain; CCR, central conserved region; TCR, terminal conserved region.
Sequencing and bioinformatic analyses
The purified PCR products were subjected to Sanger sequencing (Eurofins Genomics, Vienna, Austria) using the same GYSVd-1-specific primers employed in RT-PCR. The genomic sequences were aligned to the reference GYSVd-1 genome (GenBank accession number NC001920) and manually edited to begin at the first nucleotide position. The complete GYSVd-1 genomic sequences obtained in this study were submitted to the GenBank database and assigned accession numbers PQ858374 – PQ858402. Phylogenetic analyses and sequence comparisons were conducted using the Molecular Evolutionary Genetics Analysis software, version 10 (MEGA X) (Kumar et al., 2018). Multiple sequence alignments were performed using the ClustalW algorithm. Phylogenetic trees were constructed using the Maximum Likelihood method, applying the best-fitting nucleotide substitution model as determined by MEGA X’s model selection tool. For the construction of trees presented in this study, the general time reversible model with gamma distributed rates among sites (GTR+G) was applied. Tree reliability was assessed with 1,500 bootstrap replicates to ensure the robustness of clustering patterns. The secondary RNA structures of Slovak GYSVd-1 isolates were reconstructed using the Mfold web server (Zuker, 2003) (available at http://www.unafold.org/mfold/applications/rna-folding-form.php). Predictions were carried out under default parameters for circular RNA molecules.
Cloning
To analyze the intrahost variability of GYSVd-1, selected PCR products displaying mixed chromatogram peaks were ligated into the pGEM-T Easy vector system (Promega, Madison, WI, United States) following the manufacturer’s protocol, enabling the isolation of individual subclones. Competent Escherichia coli JM109 cells were transformed with the ligation products and plated on LB agar containing carbenicillin, X-Gal, and IPTG for blue-white screening. White colonies were selected and grown in LB broth for plasmid extraction. Purified plasmids were digested with FastDigest EcoRI restriction enzyme (Thermo Fisher Scientific, Waltham, MA, United States) followed by agarose gel electrophoresis to verify the presence and size of cloned PCR product. Individual plasmid subclones (3–10 per isolate) carrying the insert of expected size were sequenced using pUC/M13 primers (Promega, Madison, WI, United States). Complete GYSVd-1 sequences obtained by subcloning were submitted to the GenBank database and assigned accession numbers PQ858403 – PQ858424.
Results and discussion
Prevalence of GYSVd-1 in Slovak vineyards
Grapevine samples were collected from several vineyards across western and southern Slovakia, representing diverse geographical locations and grapevine cultivars. Within individual vineyards, multiple white and blue-berries varieties (both known and unknown) were sampled to account for the different genetic origins of the plant material. None of the tested plants exhibited visible symptoms of yellow speckle disease, consistent with the often asymptomatic nature of GYSVd-1 infections under certain conditions (Hadidi et al., 2017). Some of the sampled plants exhibited mild, non-specific symptoms, which could be attributed to physiological disorders or an underlying viral etiology. However, the samples were not analyzed for the presence of other viral or subviral grapevine pathogens, which could potentially explain the observed symptomatology.
Out of the 49 grapevine samples analyzed, 36 were tested positive for the presence of GYSVd-1, yielding an overall prevalence of approximately 73%. The prevalence rates varied more between vineyards rather than across geographical locations, suggesting that the primary mode of viroid dissemination is through vegetative propagation of infected source material. Such a high infection rate aligns with findings from previous studies. Despite using different methodology and primer pairs for RT-PCR detection, a survey conducted in Italy reported GYSVd-1 infection in 82.7% of analyzed samples, with the viroid detected across most cultivars regardless of geographical location. Additionally, GYSVd-1 was identified in four out of 27 tested rootstocks (14.8%) (Gambino et al., 2014). Similarly, studies from Australia, where GYSVd-1 was first reported, showed its exceptionally high prevalence. In a survey of 32 grapevine samples, all were tested positive for GYSVd-1, although only eight displayed symptoms of yellow speckle disease (Salman et al., 2014). In contrast, similar studies conducted in other regions reported significantly lower prevalence rates of GYSVd-1. For instance, surveys in Turkey found infection rates ranging from 0.09%–12.5% of analyzed samples (Gazel and Önelge, 2003; Gökçek and Önelge, 2007), while a study in Nigeria observed a prevalence of 16% (Zongoma et al., 2018). In South Africa, the reported infection rate reached 46.3% (Morgan et al., 2023), which, while notable, remains lower than the rates observed in Slovakia, Italy, or Australia. These findings suggest the difference in GYSVd-1 prevalence across different parts of the world, likely influenced by factors such as climate, vineyard management practices, and the origin of planting material. It is important to emphasize that the grapevine is grown as a double-component system, comprising a rootstock and a grafted variety, each of which may originate from different geographical regions and potentially serve as carriers of phytopathogens. Consequently, interpreting results related to pathogen diversity or introduction pathways can be challenging. It should be also noted that the prevalence data may be affected by the limited pool of tested samples and the sensitivity of the detection method used. Indeed, previous study has shown that certain GYSVd-1 variants may escape detection in multiplex assays due to sequence variability in genomic regions where GYSVd-1-specific primers are designed (Gambino et al., 2014).
Sequencing of the complete GYSVd-1 genomes revealed that 29 samples produced clean chromatograms, enabling reliable and straightforward determination of their sequences (Table 1). In contrast, seven isolates exhibited mixed chromatogram peaks, indicative of intrahost GYSVd-1 variability (Table 2). These samples therefore required additional analyses via subcloning to resolve individual sequence variants present in the viroid population and further investigate the population structure of GYSVd-1 within a single host.
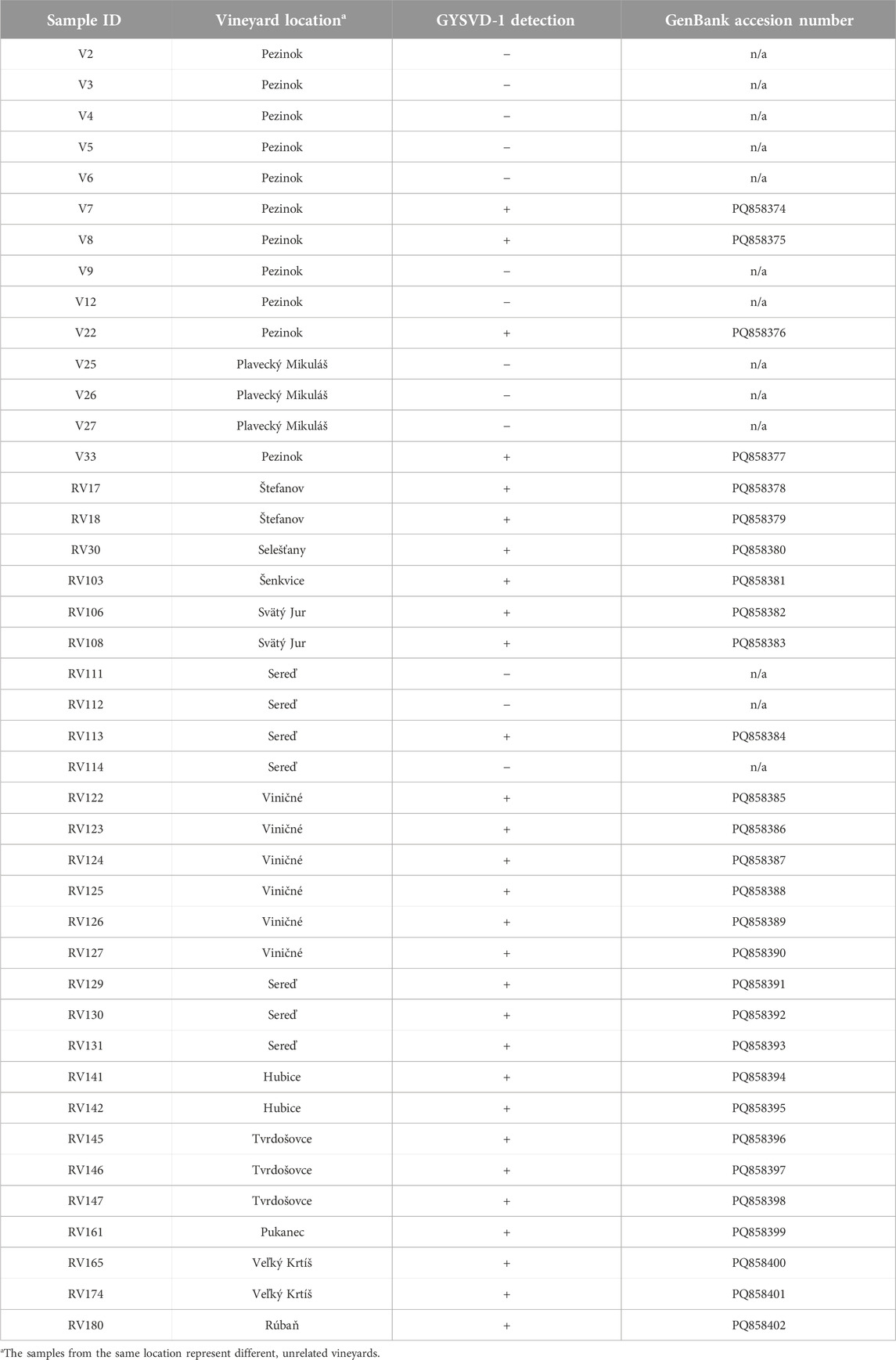
Table 1. Summary of grapevine samples tested for GYSVd-1 in Slovak vineyards, exhibiting clean chromatograms. The table lists each sample’s ID, vineyard location, GYSVd-1 detection results and the GenBank accession number assigned (if applicable).
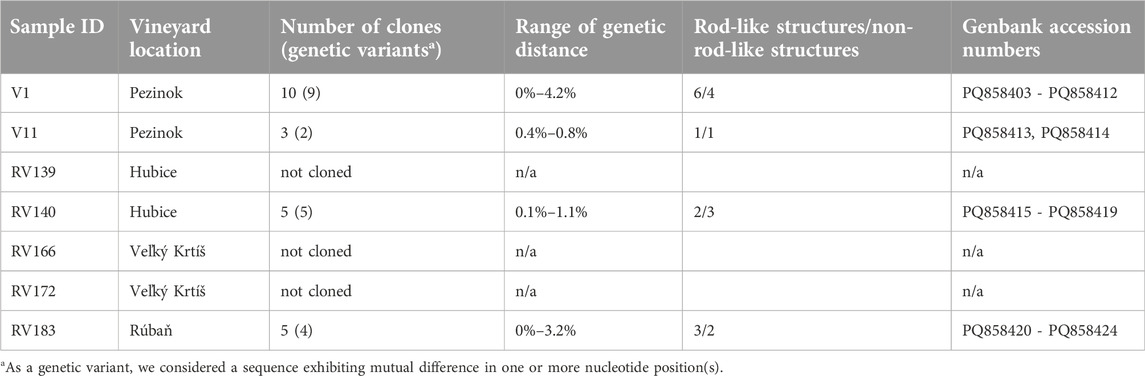
Table 2. Summary of GYSVd-1 samples exhibiting ambiguous sequence. The table includes sample ID, vineyard location, number of clones analyzed, genetic variants identified, range of genetic distances observed, number of variants exhibiting rod-like/non-rod-like secondary RNA structure and corresponding GenBank accession numbers of subclones (if applicable).
Molecular characterization of Slovak GYSVd-1 isolates
Following the sequencing of RT-PCR-amplified GYSVd-1 genomes, only isolates yielding unambiguous sequence data were included in the preliminary molecular analyses. This approach ensured that the sequences accurately represented individual plants without interference from mixed populations of viroid variants, which were identified in some samples by the presence of overlapping chromatogram peaks. Molecular characterization of Slovak GYSVd-1 isolates revealed a high level of mutual sequence identity among the isolates, ranging from 96.2% to 100%, with a mean nucleotide distance of 1.9% (s.e. 0.5%). This degree of sequence conservation is consistent with previous studies, which reported low variability within GYSVd-1 populations (Sahana et al., 2013; Fajardo et al., 2016; Vozárová et al., 2016; Morgan et al., 2023). The observed genetic similarity suggests limited divergence among the Slovak isolates, likely due to the vegetative propagation of regional grapevine cultivars and rootstocks established in our conditions, which restricts the introduction of distant genetic variants. Despite the high sequence identity, minor variations were identified, indicating ongoing evolutionary processes such as mutation and selection within the viroid genome. Given the non-coding nature of viroids and their major reliance on secondary structure (López-Carrasco and Flores, 2017), even subtle variations in nucleotide sequence could lead to structural changes that may influence the biological and epidemiological behavior of such isolate, including its adaptability and interactions with host plants and environmental factors. Sequence analysis of all Slovak GYSVd-1 isolates revealed several nucleotide diversity hotspots within the genome, suggesting that these regions are subject to less selection pressure compared to the more conserved domains (Figure 1).
Previous studies identified four distinct phylogenetic groups (types) of GYSVd-1, reflecting its global genetic diversity (Fajardo et al., 2016). Our analysis revealed that Slovak GYSVd-1 isolates were distributed randomly among various isolates from the GenBank database, with no clear association to any specific phylogenetic group (Figure 2). These results align with our previous findings (Vozárová et al., 2016). Lack of unique regional clustering among isolates was already demonstrated in previous studies (Fajardo et al., 2016; Tangkanchanapas et al., 2017; Morgan et al., 2023) and could result from the widespread exchange and propagation of grapevine planting material across international borders.
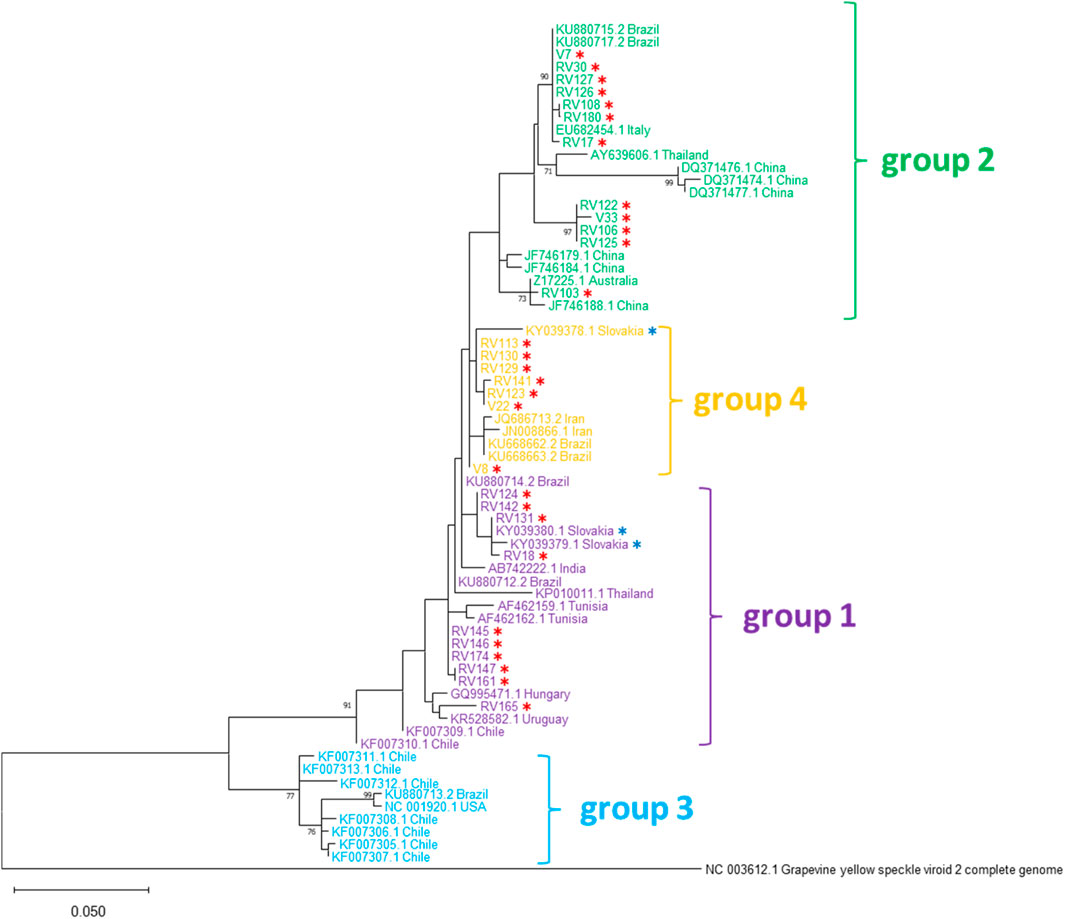
Figure 2. Phylogenetic tree based on the alignment of nucleotide sequences of 29 Slovak GYSVd-1 isolates from this study (marked with red asterisks), three Slovak isolates previously described by Vozárová et al. (2016) (marked with blue asterisks) and isolates from other countries. The phylogenetic tree was constructed using the Maximum Likelihood method with the General Time Reversible (GTR) model and gamma-distributed rates among sites (GTR+G) in MEGA X software and 1,500 bootstrap replications. GenBank accession numbers, country of origin, and previously established phylogenetic groups are indicated. Grapevine yellow speckle viroid 2 (GYSVd-2) was used as an outgroup.
However, intraspecific phylogenetic groupings of viroids like GYSVd-1 should be approached with caution, as the observed patterns, such as clustering, may be random or misleading. Viroids, as non-coding agents, are subject to constant mutations, forming quasispecies that rely on maintaining their RNA secondary structure (Ambrós et al., 1998). Due to convergent evolution, shared nucleotide sequence motifs or mutations in different isolates may not reflect a common origin but rather arise independently as compensatory changes to preserve structural integrity. Especially in the case of rod-like RNA secondary structures, mutations that alter essential structural features are often compensated by additional changes in the opposite strand to maintain the proper secondary structure required for functionality. This structural interdependence amplifies the accumulation of mutations, as a single structure-altering nucleotide change often necessitates a second compensatory mutation. Consequently, traditional phylogenetic analyses based solely on nucleotide similarity may oversimplify the complex evolutionary dynamics of viroids and fail to account for these structural constraints. This hypothesis is further supported by previous study on hop stunt viroid (HSVd), which also demonstrated this strong correlation of some specific mutations with the compensatory effect in certain positions (Alaxin et al., 2023). Exploring structure-based evolutionary models could yield more meaningful interpretations of observed patterns. Previous studies have already suggested that incorporating RNA secondary structure analyses can significantly enhance the accuracy and robustness of phylogenetic tree reconstruction (Telford et al., 2005; Grajales et al., 2007; Keller et al., 2010). Therefore, considering RNA secondary structure in non-coding RNAs could provide deeper insights into phylogenetic relationships and evolutionary dynamics.
Intrahost variability of GYSVd-1
Four of the seven samples exhibiting ambiguous sequence data were subcloned to determine the sequences of individual viroid variants within the mixed populations. Analysis of the resultant subclones revealed nucleotide identity ranging from 95.8% to 100%, with a mean nucleotide distance of 2.2% (s.e. 0.4%). Similar variability among GYSVd-1 isolates within a single host has been demonstrated in previous studies (Rigden and Rezaian, 1993; Szychowski et al., 1998). These findings suggest that intrahost variability is comparable to the variability observed among isolates derived from different plants. This indicates that the dynamics of GYSVd-1 populations within a single host are shaped by similar evolutionary pressures as those acting at the interhost level, likely driven by the maintenance of structural stability crucial for viroid functionality. This is consistent with studies indicating that viroid populations accumulate in infected plants as quasispecies–a group of closely related sequences subjected to continuous mutation and selection–within individual hosts (Ambrós et al., 1998; Codoñer et al., 2006). This variability originates from the error-prone nature of viroid replication, which introduces frequent mutations (Flores et al., 2014). However, the sequence diversity is not unlimited, as structural and functional constraints ensure the preservation of essential elements, such as the central conserved region (CCR) and the characteristic rod-like secondary structure in members of the family Pospiviroidae, as suggested by recent study (Nie et al., 2023). Furthermore, host-specific and even tissue-specific selection pressures may also influence the composition of viroid populations (Flores et al., 2020). Phylogenetic analyses of individual GYSVd-1 clones revealed no significant relationships among them (Supplementary Figure S1), indicating that the observed mutations and polymorphisms occur randomly. Since the total RNA was isolated from three leaves sampled from different parts of a single plant, it is not possible to determine whether the observed intra-host variability arises from multiple viroid populations co-existing within a single leaf or if it results from spatial separation of distinct populations across different leaves within the same plant. Understanding whether variants are evenly distributed throughout the plant or instead form spatially separated subpopulations could improve sampling strategies for diagnostics and our understanding of viroid movement within the plant. However, previously published studies have demonstrated the presence of multiple viroid sequence variants within a single cell, a single leaf, or across different tissue types (Wu and Bisaro, 2024; Serra et al., 2023; Chambers et al., 2024). Viroid variants co-existing within a single cell or tissue could interact in ways that either enhance or suppress each other’s replication or pathogenic effects. Indeed, as demonstrated in a recent study, chrysathemum chlorotic spot viroid (CChMVd) displays a “territorial behavior,” where certain sequence variants can dominate specific leaf sectors, effectively excluding other variants through a mechanism referred to as superinfection exclusion. Such exclusion dynamics, coupled with bottleneck effects during viroid movement and colonization, contribute to the spatial segregation of variant populations within infected tissues (Serra et al., 2023).
Reconstruction and analysis of secondary RNA structures in Slovak GYSVd-1 isolates
The secondary RNA structures of Slovak GYSVd-1 isolates were reconstructed to assess their structural integrity and biological relevance. All unambiguous GYSVd-1 isolates exhibited the characteristic rod-like secondary structure typical for viroids in the family Pospiviroidae. We considered a sequence to be biologically relevant if a proper rod-like structure was among the five most stable predicted RNA conformations, as determined by Gibbs free energy values. In contrast, a substantial proportion of GYSVd-1 clones derived from samples exhibiting mixed chromatogram failed to form proper rod-like conformations (Table 2). This structural instability suggests that some variants within mixed populations may lack functional viability, potentially representing replication by-products or mutants unable to sustain independent replication.
Forming a proper secondary and even tertiary structure is assumed to be crucial for viroid replication and interaction with host factors, as demonstrated by previous studies (Zhong et al., 2008; Ma and Wang, 2022; Nie et al., 2023). Unlike viruses, viroids lack ORFs, making it impossible to identify defective variants through typical indicators such as translational frameshifts or premature stop codons. Consequently, verifying the secondary structure of viroid sequences may be helpful to differentiate functional variants from defective ones. Our hypothesis is supported by previous findings, such as a study on Potato spindle tuber viroid (PSTVd) that evaluated the infectivity of isolates with rod-like and non-rod-like structures. The study demonstrated that isolates with rod-like conformations successfully established infections in all tested plants, whereas those with non-rod-like structures either failed to infect or exhibited significantly reduced infection rates, suggesting that the rod-like structure is a prerequisite for the successful infection of PSTVd. Interestingly, this study demonstrated that increasing the amount of RNA transcripts led to an enhanced infection rate in all three non-rod-like forms, suggesting the existence of additional RNA secondary structures, such as the correct rod-like structure, alongside the dominant structure in the RNA inoculum of these forms (Nie et al., 2023). Based on these data, precautions should be taken to thoroughly evaluate sequence data and to predict secondary structures using reliable tools. Variants exhibiting aberrant structures should be carefully noted, as these could represent nonviable by-products of replication. Moreover, when submitting viroid sequences to public databases, researchers should include annotations or comments regarding any defective structures observed to ensure accurate interpretation and to aid future studies on viroid evolution and functionality.
We further analyzed the secondary RNA structures of various GYSVd-1 sequences deposited in GenBank to evaluate their structural integrity. As expected, several of these sequences exhibited defective RNA structures, further confirming the pattern observed in our study. However, the predicted RNA structures could be affected by sequence accuracy, as errors introduced during RT-PCR and sequencing–such as base miscalls or untrimmed artifacts–can lead to incorrect structural predictions. Therefore, it is crucial to exercise serious precautions during the processing and analysis of sequence data. This includes proper trimming of sequences, careful verification of chromatogram peaks, and ensuring that only high-quality, unambiguous sequences are used for structural reconstruction.
All predicted rod-like secondary structures of GYSVd-1 isolates and clones were compared to assess structural variability. The most significant differences were observed in the pathogenicity domain (Figure 3), suggesting potential functional implications. This domain, while not solely responsible, is thought to be associated with symptom severity (Schnölzer et al., 1985), indicating that structural variations in this region might influence the biological behavior of the viroid. In contrast, the left terminal domain remained structurally conserved across all analyzed isolates. This finding aligns with previous studies highlighting the critical role of this region in viroid replication, as it serves as the initiation site for RNA polymerase II (Kolonko et al., 2006; Bojić et al., 2012).
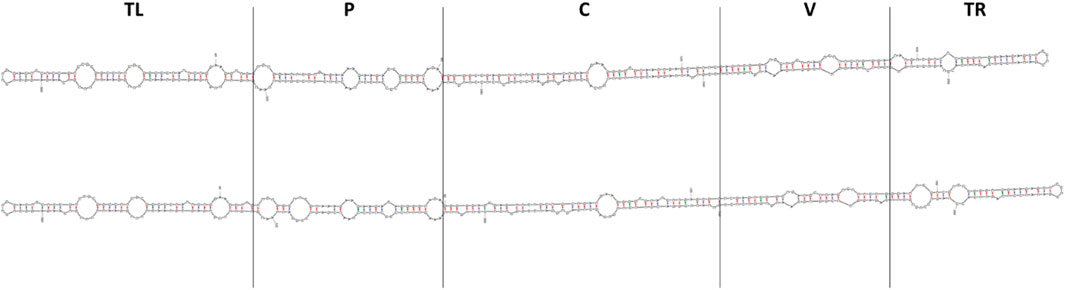
Figure 3. Comparison of predicted secondary RNA structures of the two most distant GYSVd-1 isolates obtained in this study. Isolate V33 (top) and isolate RV18 (bottom) shared 96.2% nucleotide identity, giving a genetic distance of 3.8%. The highest structural variability was observed in the pathogenicity domain, while the left terminal domain remained conserved across all analyzed isolates. Structures were predicted using mFold RNA folding form (Zuker, 2003) and default settings for circular RNA molecules. TL, terminal left domain; P, pathogenicity domain; C, central domain; V, variable domain; TR, terminal right domain.
Conclusion
This study provides valuable insights into the prevalence and molecular diversity of GYSVd-1 in Slovakia, where viticulture represents a significant agricultural sector. The detection of GYSVd-1 in 73% of tested samples highlights its high prevalence in the region, emphasizing the need for continued monitoring and management of viroid infections in vineyards. The lack of observable symptoms in infected plants further underscores the viroid’s cryptic nature and its potential to persist undetected, posing a risk of widespread dissemination through vegetative propagation. The symptoms associated with GYSVd-1 infection are often challenging to determine due to the frequent co-infection with other plant pathogens or the presence of physiological disorders. These overlapping factors can obscure the specific symptoms of GYSVd-1 presence, thus complicating diagnosis and understanding of its role in plant health. Molecular characterization revealed a high degree of sequence identity among Slovak GYSVd-1 isolates, with a mean nucleotide divergence of 1.9%. While this suggests limited genetic variation, the detection of subtle mutations indicates ongoing evolutionary processes. Phylogenetic analyses demonstrated that Slovak isolates are distributed among various global phylogenetic groups, supporting the hypothesis that geographic location does not dictate intraspecific grouping. However, the random distribution of isolates within phylogenetic trees and the occurrence of convergent mutations emphasize the limitations of traditional phylogenetic methods for understanding intraspecific evolutionary relations in the case of non-coding subviral elements, such as viroids. The observation of intrahost variability in several isolates further supports the concept of viroids as quasispecies, with populations shaped by continuous mutation and selection. This variability, comparable to interhost variability, underscores the complex dynamics of viroid populations and the role of structural and functional constraints in maintaining critical RNA elements. Finally, this study highlights the importance of RNA secondary structure in determining the biological relevance of GYSVd-1 isolates. While unambiguous isolates consistently exhibited the characteristic rod-like structure essential for viroid replication, many variants from mixed populations showed defective conformations, indicating potential nonviability. These findings emphasize the need for careful structural verification in viroid analyses to differentiate functional variants from replication by-products and ensure accurate interpretation of viroid diversity and evolution.
Our findings contribute to the growing body of knowledge on GYSVd-1 epidemiology and molecular evolution, with practical implications for viticulture and data interpretation. Monitoring viroid populations, understanding their genetic variability, and identifying pathways of dissemination are essential for developing targeted management strategies to minimize the impact of GYSVd-1 on grapevine health and productivity. Moreover, advancing our understanding of the evolutionary mechanisms governing viroid diversity will contribute to future studies on the biology and control of non-coding RNA pathogens.
Data availability statement
The datasets presented in this study can be found in online repositories. The names of the repository/repositories and accession number(s) can be found below: https://www.ncbi.nlm.nih.gov/genbank/, PQ858374-PQ858424.
Author contributions
Conceptualization, MG and AA; methodology, JK, PA, MG, and AA; investigation, JK, PA, LP, ZŠ, MG, and AA; resources, MG and AA; data curation, MG and AA; writing - original draft preparation, MG and AA; writing - review and editing, JK, PA, LP, ZŠ, MG, and AA; funding acquisition, MG. All authors contributed to the article and approved the submitted version.
Funding
The author(s) declare that financial support was received for the research and/or publication of this article. This research was supported by grant APVV-22-0067 from the Slovak Research and Development Agency. JK was funded by the EU NextGenerationEU through the Recovery and Resilience Plan for Slovakia under the project No. 09I03-03-V05-00004.
Conflict of interest
The authors declare that the research was conducted in the absence of any commercial or financial relationships that could be construed as a potential conflict of interest.
Generative AI statement
The author(s) declare that no Generative AI was used in the creation of this manuscript.
Supplementary material
The Supplementary Material for this article can be found online at: https://www.frontierspartnerships.org/articles/10.3389/av.2025.14361/full#supplementary-material
Footnotes
1Accessed from https://ictv.global/report/chapter/pospiviroidae/pospiviroidae/apscaviroid on 24th November 2024.
References
Abdullah, A. S., Moffat, C. S., Lopez-Ruiz, F. J., Gibberd, M. R., Hamblin, J., and Zerihun, A. (2017). Host-multi-pathogen warfare: pathogen interactions in Co-infected plants. Front. Plant Sci. 8, 1806. doi:10.3389/fpls.2017.01806
Alaxin, P., Predajňa, L., Achs, A., Šubr, Z., Mrkvová, M., and Glasa, M. (2023). Analysis of hop stunt viroid diversity in grapevine (Vitis vinifera L.) in Slovakia: coexistence of two particular genetic groups. 2023. Pathogens 12 (2), 205. doi:10.3390/pathogens12020205
Ambrós, S., Hernández, C., Desvignes, J. C., and Flores, R. (1998). Genomic structure of three phenotypically different isolates of peach latent mosaic viroid: Implications of the existence of constraints limiting the heterogeneity of viroid quasispecies. J. Virology 72 (9), 7397–7406. doi:10.1128/JVI.72.9.7397-7406.1998
Bojić, T., Beeharry, Y., Zhang, D. J., and Pelchat, M. (2012). Tomato RNA polymerase II interacts with the rod-like conformation of the left terminal domain of the potato spindle tuber viroid positive RNA genome. J. General Virology 93 (7), 1591–1600. doi:10.1099/vir.0.041574-0
Chambers, G. A., Geering, A. D. W., Bogema, D. R., Holford, P., Vidalakis, G., and Donovan, N. J. (2024). Characterisation of the genetic diversity of citrus viroid VII using amplicon sequencing. Archives Virology 170 (1), 12. doi:10.1007/s00705-024-06191-4
Codoñer, F. M., Darós, J. A., Solé, R. V., and Elena, S. F. (2006). The fittest versus the flattest: Experimental confirmation of the quasispecies effect with subviral pathogens. PLoS Pathog. 2 (12), e136. doi:10.1371/journal.ppat.0020136
Delic, D., Radulovic, M., Vukosavjevic, V., and Massart, S. (2018). First report of grapevine rupestris stem pitting-associated virus, hop stunt viroid, and grapevine yellow speckle viroid 1 infecting grapevine (Vitis vinifera) in Bosnia and Herzegovina. Plant Dis. 102 (12), 2666. doi:10.1094/PDIS-03-18-0473-PDN
Diener, T. O. (2001). The viroid: Biological oddity or evolutionary fossil? Adv. Virus Res. 57, 137–184. doi:10.1016/s0065-3527(01)57003-7
Elleuch, A., Hamdi, I., Bessaies, N., and Fakhfakh, H. (2013). Single-strand conformation polymorphism for molecular variability studies of six viroid species. Biosci. Biotechnol. Biochem. 77 (1), 182–188. doi:10.1271/bbb.120714
Fajardo, T. V. M., Eiras, M., and Nickel, O. (2016). Detection and molecular characterization of Grapevine yellow speckle viroid 1 isolates infecting grapevines in Brazil. Trop. Plant Pathol. 41, 246–253. doi:10.1007/s40858-016-0097-1
Flores, R., Daròs, J. A., Hernández, C., Navarro, B., and Di Serio, F. (2020). Viroids. eLS 1 (1), 192–203. doi:10.1002/9780470015902.a0029141
Flores, R., Gago-Zachert, S., Serra, P., Sanjuán, R., and Elena, S. F. (2014). Viroids: Survivors from the RNA world? Annu. Rev. Microbiol. 68, 395–414. doi:10.1146/annurev-micro-091313-103416
Gambino, G., Navarro, B., Torchetti, E. M., La Notte, P., Schneider, A., Mannini, F., et al. (2014). Survey on viroids infecting grapevine in Italy: Identification and characterization of Australian grapevine viroid and grapevine yellow speckle viroid 2. Eur. J. Plant Pathology 140, 199–205. doi:10.1007/s10658-014-0458-x
Gazel, M., and Önelge, N. (2003). First report of grapevine viroids in the east Mediterranean region of Turkey. Plant Pathol. 52, 405. doi:10.1046/j.1365-3059.2003.00827.x
Gökçek, B., and Önelge, B. (2007). “Investigation of grapevine yellow speckle disease (GYSVd-1 and 2) in the vineyards of Gaziantep province,” in Proceedings of the Turkey II plant protection congress, 27–29 august 2007, isparta, Turkey, 118.
Grajales, A., Aguilar, C., and Sánchez, J. A. (2007). Phylogenetic reconstruction using secondary structures of internal transcribed spacer 2 (ITS2, rDNA): Finding the molecular and morphological gap in caribbean gorgonian corals. BMC Evol. Biol. 7, 90. doi:10.1186/1471-2148-7-90
Habili, N. (2017). “Apscaviroids infecting grapevine,” in Viroids and satellites (Academic Press), 251–262.
Hadidi, A., Vidalakis, G., and Sano, T. (2017). “Economic significance of fruit tree and grapevine viroids,” in Viroids and satellites (Academic Press), 15–25.
Jo, Y., Choi, H., Song, M. K., Park, J. S., Lee, J. W., and Cho, W. K. (2017). First report of Grapevine yellow speckle viroid 1 and Hop stunt viroid infecting grapevines (Vitis vinifera) in Korea. Plant Dis. 101 (6), 1069. doi:10.1094/PDIS-12-16-1759-PDN
Keller, A., Förster, F., Müller, T., Dandekar, T., Schultz, J., and Wolf, M. (2010). Including RNA secondary structures improves accuracy and robustness in reconstruction of phylogenetic trees. Biol. Direct 5, 4. doi:10.1186/1745-6150-5-4
Kolonko, N., Bannach, O., Aschermann, K., Hu, K., Moors, M., Schmitz, M., et al. (2006). Transcription of potato spindle tuber viroid by RNA polymerase II starts in the left terminal loop. Virology 347 (2), 392–404. doi:10.1016/j.virol.2005.11.039
Koltunow, A. M., and Rezaian, M. A. (1988). Grapevine yellow speckle viroid: Structural features of a new viroid group. Nucleic Acids Res. 16 (3), 849–864. doi:10.1093/nar/16.3.849
Koltunow, A. M., Krake, L. R., Johnson, S. D., and Rezaian, M. A. (1989). Two related viroids cause grapevine yellow speckle disease independently. J. General Virology 70 (12), 3411–3419. doi:10.1099/0022-1317-70-12-3411
Kumar, S., Stecher, G., Li, M., Knyaz, C., and Tamura, K. (2018). Mega X: molecular evolutionary genetics analysis across computing platforms. Mol. Biol. Evol. 35 (6), 1547–1549. doi:10.1093/molbev/msy096
López-Carrasco, A., and Flores, R. (2017). Dissecting the secondary structure of the circular RNA of a nuclear viroid in vivo: a “naked” rod-like conformation similar but not identical to that observed in vitro. RNA Biol. 14 (8), 1046–1054. doi:10.1080/15476286.2016.1223005
Ma, J., and Wang, Y. (2022). Studies on viroid shed light on the role of RNA three-dimensional structural motifs in RNA trafficking in plants. Front. Plant Sci. 13, 836267. doi:10.3389/fpls.2022.836267
Martelli, G. P. (2014). Directory of virus and virus-like diseases of the grapevine and their agents. J. Plant Pathology 96 (1), 1–136.
Morgan, S. W., Read, D. A., Burger, J. T., and Pietersen, G. (2023). Diversity of viroids infecting grapevines in the South African Vitis germplasm collection. Virus Genes 59 (2), 244–253. doi:10.1007/s11262-023-01971-7
Nie, Y., Zhang, Y., and Wu, J. (2023). The secondary structure of potato spindle tuber viroid determines its infectivity in Nicotiana benthamiana. Viruses 15 (12), 2307. doi:10.3390/v15122307
Rigden, J. E., and Rezaian, M. A. (1993). Analysis of sequence variation in grapevine yellow speckle viroid 1 reveals two distinct alternative structures for the pathogenic domain. Virology 193 (1), 474–477. doi:10.1016/s0042-6822(15)80001-4
Sahana, A. B., Adkar-Purushothama, C. R., Chennappa, G., Zhang, Z. X., Sreenivasa, M. Y., and Sano, T. (2013). First report of grapevine yellow speckle viroid-1 and hop stunt viroid infecting grapevines (Vitis vinifera) in India. Plant Dis. 97 (11), 1517. doi:10.1094/PDIS-05-13-0494-PDN
Salman, T. M., Habili, N., and Shi, B. (2014). Effect of temperature on symptom expression and sequence polymorphism of grapevine yellow speckle viroid 1 in grapevine. Virus Res. 189, 243–247. doi:10.1016/j.virusres.2014.03.028
Schnölzer, M., Haas, B., Raam, K., Hofmann, H., and Sänger, H. L. (1985). Correlation between structure and pathogenicity of potato spindle tuber viroid (PSTV). EMBO J. 4 (9), 2181–2190. doi:10.1002/j.1460-2075.1985.tb03913.x
Serra, P., Navarro, B., Forment, J., Gisel, A., Gago-Zachert, S., Di Serio, F., et al. (2023). Expression of symptoms elicited by a hammerhead viroid through RNA silencing is related to population bottlenecks in the infected host. New Phytol. 239 (1), 240–254. doi:10.1111/nph.18934
Szychowski, J. A., Credi, R., Reanwarakorn, K., and Semancik, J. S. (1998). Population diversity in grapevine yellow speckle viroid-1 and the relationship to disease expression. Virology 248 (2), 432–444. doi:10.1006/viro.1998.9292
Tangkanchanapas, P., Reanwarakorn, K., Juenak, H., and De Jonghe, K. (2017). First report of Grapevine yellow speckle viroid-2 infecting grapevine (Vitis vinifera) in Thailand. New Dis. Rep. 36 (1), 6. doi:10.5197/j.2044-0588.2017.036.006
Taylor, R. H., and Woodham, R. C. (1972). Grapevine yellow speckle — a newly recognized graft-transmissible disease of Vitis. Aust. J. Agric. Res. 23 (3), 447–452. doi:10.1071/AR9720447
Telford, M. J., Wise, M. J., and Gowri-Shankar, V. (2005). Consideration of RNA secondary structure significantly improves likelihood-based estimates of phylogeny: Examples from the bilateria. Mol. Biol. Evol. 22 (4), 1129–1136. doi:10.1093/molbev/msi099
Vozárová, Z., Sihelská, N., Predajňa, L., Šoltys, K., and Glasa, M. (2016). First report of grapevine yellow speckle viroid-1 infecting grapevines in Slovakia. J. Plant Pathology 98 (3), 697. doi:10.4454/jpp.v98i3.3770
Ward, L. I., Burnip, G. M., Liefting, L. W., Harper, S. J., and Clover, G. R. G. (2011). First Report of Grapevine yellow speckle viroid 1 and Hop stunt viroid in Grapevine (Vitis vinifera) in New Zealand. Plant Dis. 95 (5), 617. doi:10.1094/PDIS-12-10-0927
Wu, J., and Bisaro, D. M. (2024). Potato spindle tuber viroid (PSTVd) loop 27 mutants promote cell-to-cell movement and phloem unloading of the wild type: insights into RNA-based viroid interactions. Virology 597, 110137. doi:10.1016/j.virol.2024.110137
Zhong, X., Archual, A. J., Amin, A. A., and Ding, B. (2008). A genomic map of viroid RNA motifs critical for replication and systemic trafficking. Plant Cell 20 (1), 35–47. doi:10.1105/tpc.107.056606
Zongoma, A. M., Dangora, D. B., Al Rwahnih, M., Bako, S. P., Alegbejo, M. D., and Alabi, O. J. (2018). First Report of Grapevine yellow speckle viroid 1, Grapevine yellow speckle viroid 2, and Hop stunt viroid Infecting Grapevines (Vitis spp.) in Nigeria. Plant Dis. 102 (1), 259. doi:10.1094/PDIS-07-17-1133-PDN
Keywords: Apscaviroid alphaflavivitis, detection, epidemiology, molecular diversity, Vitis vinifera
Citation: Kemenczeiová J, Alaxin P, Predajňa L, Šubr Z, Glasa M and Achs A (2025) High prevalence and low genetic diversity of grapevine yellow speckle viroid 1 in Slovakia: down the rabbit hole of RNA secondary structure and phylogeny. Acta Virol. 69:14361. doi: 10.3389/av.2025.14361
Received: 17 January 2025; Accepted: 31 March 2025;
Published: 08 April 2025.
Edited by:
Katarina Polcicova, Slovak Academy of Sciences, SlovakiaReviewed by:
Çiğdem Ulubaş Serçe, Niğde Ömer Halisdemir University, TürkiyeDana Šafářová, Palacký University Olomouc, Czechia
Copyright © 2025 Kemenczeiová, Alaxin, Predajňa, Šubr, Glasa and Achs. This is an open-access article distributed under the terms of the Creative Commons Attribution License (CC BY). The use, distribution or reproduction in other forums is permitted, provided the original author(s) and the copyright owner(s) are credited and that the original publication in this journal is cited, in accordance with accepted academic practice. No use, distribution or reproduction is permitted which does not comply with these terms.
*Correspondence: Adam Achs, YWRhbS5hY2hzQHNhdmJhLnNr