- 1Soil Science and Agricultural Chemistry, Faculty of Science, University of Vigo, Ourense, Spain
- 2Department of Soil Science and Agricultural Chemistry, Engineering Polytechnic School, University of Santiago de Compostela, Lugo, Spain
This work is focused on the study of the dissipation of the antibiotics amoxicillin (AMX) (an amino penicillin) and azithromycin (AZT) (belonging to the macrolide group), performed at a laboratory scale, under simulated sunlight and in the dark, at pH values 4.0, 5.5, and 7.2, and in the presence of humic acids and different inorganic salts. The results indicate that AMX is more affected than AZT by simulated sunlight, with half-lives ranging 7.7–9.9 h for AMX and 250–456 h for AZT. The lowest half-life values were obtained at pH 7.2 for AMX (7.7 h) and at pH 4.0 for AZT (250 h). Regarding the effect of various salts, the presence of NaNO3 causes that C/C0 decreases from 0.6 to 0 under simulated sunlight, having no effect on the dissipation of AMX in the dark. However, in the presence of FeCl3 at concentrations of 500 mg L−1, AMX suffered total dissipation, both under simulated sunlight and in the dark. For AZT the influence was lower, and the salts that caused a higher increase in its dissipation were NaCl (with C/C0 decreasing from 0.5 to 0.2) and CaCl2 (C/C0 decreasing from 0.5 to 0.3). The presence of humic acids caused a slight increase in the dissipation of AMX, both under simulated sunlight and in the dark, a fact that was attributed to the adsorption of the antibiotic onto these organic substances, which, however, caused a more marked increase in the dissipation of AZT (reaching 68%) under simulated sunlight. The overall results of this research can be considered clearly relevant, mainly to determine the fate of AMX and AZT when these antibiotics reach the environment as pollutants, either as regards their exposure to natural sunlight, or in relation to the use of inactivation/photo-degradation systems in decontamination procedures focused on environmental compartments.
Introduction
Antibiotics reaching environmental compartments are considered emerging pollutants, and its presence in soils can cause a variety of public health and environmental issues (Tello et al., 2012; Manyi-Loh et al., 2018; Iwu et al., 2020; Serwecińska, 2020). The passage of antibiotics from soils to surface-, subsurface- and ground-water (and its direct arrival to riverbeds through wastewater), as well as their entry into the food chain through crops or aquatic organisms, is considered a particularly relevant risk (Zhao et al., 2019; Kumar et al., 2020; Muhammad et al., 2020; Hassan et al., 2021). But a particular aspect, specific to the soil, is the potential harmful effects of antibiotics on edaphic microorganisms (Brandt et al., 2015; Cycoń et al., 2019; Santás-Miguel et al., 2020), both due to the direct toxicity on these microorganisms, and to the development of pathogenic antibiotic resistant bacteria (Forsberg et al., 2012; Cycoń et al., 2019). In this regard, as indicated in Salam and Obayori (2019), antibiotic resistance is common in the environment, and the meta-genomic approach has recently shown its increasing relevance, mainly in cultivated soils and environmental compartments affected by anthropogenic activities.
One of the main routes for antibiotics to reach soils and the overall environment is through wastewater and sewage sludge spreading, and since many treatment plants (WWTPs) have low efficiency in removing these compounds (Wang and Wang., 2016; Yang et al., 2016), different additional methods have been tested to inactivate/remove them, among which oxidation processes, adsorption or biological treatments stand out (Han et al., 2019; Han et al., 2021; Nippes et al., 2021). This low purification efficiency reached in WWTPs, is one of the reasons why relevant concentrations of antibiotics appear in both surface and groundwater, and even in drinking water (Kemper, 2008).
Once these antibiotics reach watercourses, their degrees of susceptibility to photo-degradation are of main importance to establishing their persistence, as occurs in soils and other environmental compartments (Cycoń et al., 2019; Nnadozie and Odume, 2019; Arun et al., 2020). This susceptibility to photo-degradation can be studied in the laboratory under simulated sunlight, allowing to determine the persistence of the antibiotics in the environment (particularly in aqueous media), which affects their probability of generating environmental and public health issues (Bilal et al., 2020; Chaturvedi et al., 2021).
Given the complexity and importance of this problem, it would be clearly interesting to obtain new experimental data regarding the degradation of antibiotics that takes place in aqueous media (which could correspond to watercourses, but could also be representative of the soil solution). In a latter step, this would help in modeling the behavior of these compounds in a variety of environmental situations, allowing to predict and fight related pollution and public health issues (Rico et al., 2019; Su et al., 2019; Tang et al., 2019; Khan et al., 2020; Bavumiragira et al., 2022).
In addition, this kind of information on photo-degradation, obtained through laboratory experiments, could be useful to find the most favorable conditions promoting the removal/inactivation of antibiotics present in wastewater, which could be studied in later phases, at pilot scale, before entering the real world scale if justified (Liu et al., 2018; Almansba et al., 2021; Silva et al., 2021).
With the above background in mind, this work was conceived to study the laboratory photo-degradation of two antibiotics: amoxicillin (AMX), a semi-synthetic derivative of penicillin that is among the most widely used antibiotic substances, and has been detected in waters of different countries (Tran et al., 2019; Sodhi et al., 2021; Yang et al., 2021), and azithromycin (AZT), a macrolide which was also detected in different water samples (Yi et al., 2019; Yang et al., 2021). This is the first study on the photo-degradation of both antibiotics in aqueous media in which the pH is modified, as well as the concentrations of different salts and humic acids. The results of this research could shed light on these processes, which are highly relevant at the level of different environmental compartments, public health and the overall environment.
Materials and Methods
Chemicals and Reagents
The antibiotics used were Amoxicillin tri-hydrate (AMX) with 95% purity, and Azithromycin (AZT), with 98% purity, both from by Sigma Aldrich (Barcelona, Spain). MilliQ water was used to prepare the AMX solution (at 50 µM), while AZT was prepared at 5 mM in 96% ethanol, and from this a 50 µM concentration was obtained by dilution with MilliQ water. All the reagents used for HPLC analyses (acetonitrile, phosphoric acid and monopotassium phosphate), and the different inorganic salts used, were of high purity analytical grade, supplied by Sigma-Aldrich (Barcelona, Spain). The most relevant physicochemical characteristics of both antibiotics are presented in Table 1.
Antibiotics Quantification
The equipment used for the quantification of the antibiotics was an UltiMate 3000 HPLC (Thermo Fisher Scientific, United States), with a quaternary pump, an auto-sampler, a thermosstatted column compartment and an UltiMate 3000 series ultraviolet-visible detector. This equipment contains a computer with version 7 of the Chromeleon software (Thermo Fisher Scientific, United States). Chromatographic separations were performed on a Luna C18 analytical column (150 mm long; 4.6 mm internal diameter; 5 µm particle size) from Phenomenex (Madrid, Spain), and a security column (4 mm long; 3 mm internal diameter; 5 µm particle size), packed with the same material as the column.
As the quantification limits were 0.01 µM for AMX and 0.5 µM for AZT, the injection volumes were lower for the former (50 µL), while it was 200 µL for AZT. The flow rates were 1.5 ml min−1 for AMX and 1 ml min−1 for AZT. The temperature was kept constant at 25 ± 1°C throughout the analysis. Finally, it should be noted that between each measurement, the syringe was washed with a methanol:water solution (ratio 50:50).
The conditions used to achieve the separation of the antibiotic AZT were the following: the mobile phase was formed by acetonitrile (phase A), and by monopotassium phosphate 0.025 M (phase B). The linear gradient elution program was executed from 5 to 70% for phase A and from 95 to 30% for phase B with a time of 18 min. The initial conditions were restored in 2 min and held for 3 min. The total time for analysis was 25 min, with a retention time of 9.7 min, and the wavelength used for detection was 210 nm.
AMX was separated under the following conditions: the mobile phase was acetonitrile (phase A) as for AZT, but with phase B being 0.01 M phosphoric acid (at pH = 2). The linear gradient elution program was executed from 5 to 15% for phase A and from 95 to 85% for phase B, within 4 min. The initial conditions were restored in 2 min. The total analysis time was 10 min, with a retention time of 3.3 min, and the wavelength used was 230 nm.
Additionally, in the aqueous solutions in which photo-degradation was studied, the total organic carbon (TOC) was determined at times of 0, 2, 16, and 72 h of exposure to light, to determine the degree of antibiotic mineralization. However, it was performed just for AMX, as AZT had been prepared in ethanol, as indicated above. Specifically, the quantification of TOC was carried out by means of a Multi N/C2100 equipment (Analytikjena, Jena, Germany).
Photo-Degradation Experiments
Influence of pH on AMX and AZT Photo-Degradation
The effect of pH on degradation was studied, both under simulated sunlight and in the dark, adjusting the solutions to three pH values (4.0, 5.5, and 7.0) with 0.5 M NaOH and 0.5 M HCl. The following conditions were used to carry out the study in the dark: Six (6) ml of the solution of each antibiotic (at concentrations of 50 µM) were placed in opaque glass EPA vials that were kept at a constant temperature (22 ± 2°C); the concentration of each antibiotic was measured at different times (between 0.1 and 72 h for AMX, and between 0.1 and 192 h for AZT) using the HPLC equipment. In the simulated sunlight experiments, the samples were placed in a Suntest CPS + Atlas cabinet (Germany) equipped with a 550 Wm−2 xenon lamp and quartz filters with a wavelength cut-off at 285 nm. The contact times were the same as those used in the experiments in the dark.
Effects of Inorganic Salts and Humic Acids on AMX and AZT Photo-Degradation
For these experiments, specific samples were prepared with a 50 µM concentration of each antibiotic and with different concentrations of each salt (0, 5, 10, 50, and 500 mg L−1) and humic acids (0, 0.05, 0.1, 0.2, 0.5, 1, and 10 mg L−1). The salts used were NaCl, CaCl2, NaNO3, Na2HPO4, and FeCl3. The solutions were adjusted to pH 4.0 with 0.5 M NaOH and 0.5 M HCl, except for the samples with humic acids, which were adjusted to pH 5.5, since these substances precipitate at more acidic pH values.
The exposure to light or dark conditions took place during a time dependent on the half-life of each antibiotic, calculated from the experimental data of degradation in water at pH 4.0, which were described with an exponential decay model presented below, being 8 h for AMX and 216 h for AZT. All experiments were performed in triplicate.
Decay Model
The experimental data were fitted to an exponential decay model (Eq. 1)
where C/C0 is the fraction of the initial concentration C0 that remains in the suspension after a given time t (expressed in min), and k (min−1) is the kinetic dissipation constant. The half-life (t1/2) of each compound was calculated as per Eq. 2:
Results
Effect of pH on AMX and AZT Photo-Degradation in Absence of Inorganic Salts and Humic Acids
Figure 1 shows the dissipation results for AMX and AZT at different pH values, both under simulated sunlight and in the dark. For AMX, there was no dissipation in the dark at the three pHs studied, keeping the value of the ratio C/C0 close to 1 (Figures 1A–C), while significant degradation occurred under simulated sunlight, although without showing a clear trend as a function of pH (Figures 1A–C), reaching C/C0 values close to 0 after 40 h of contact/exposure.
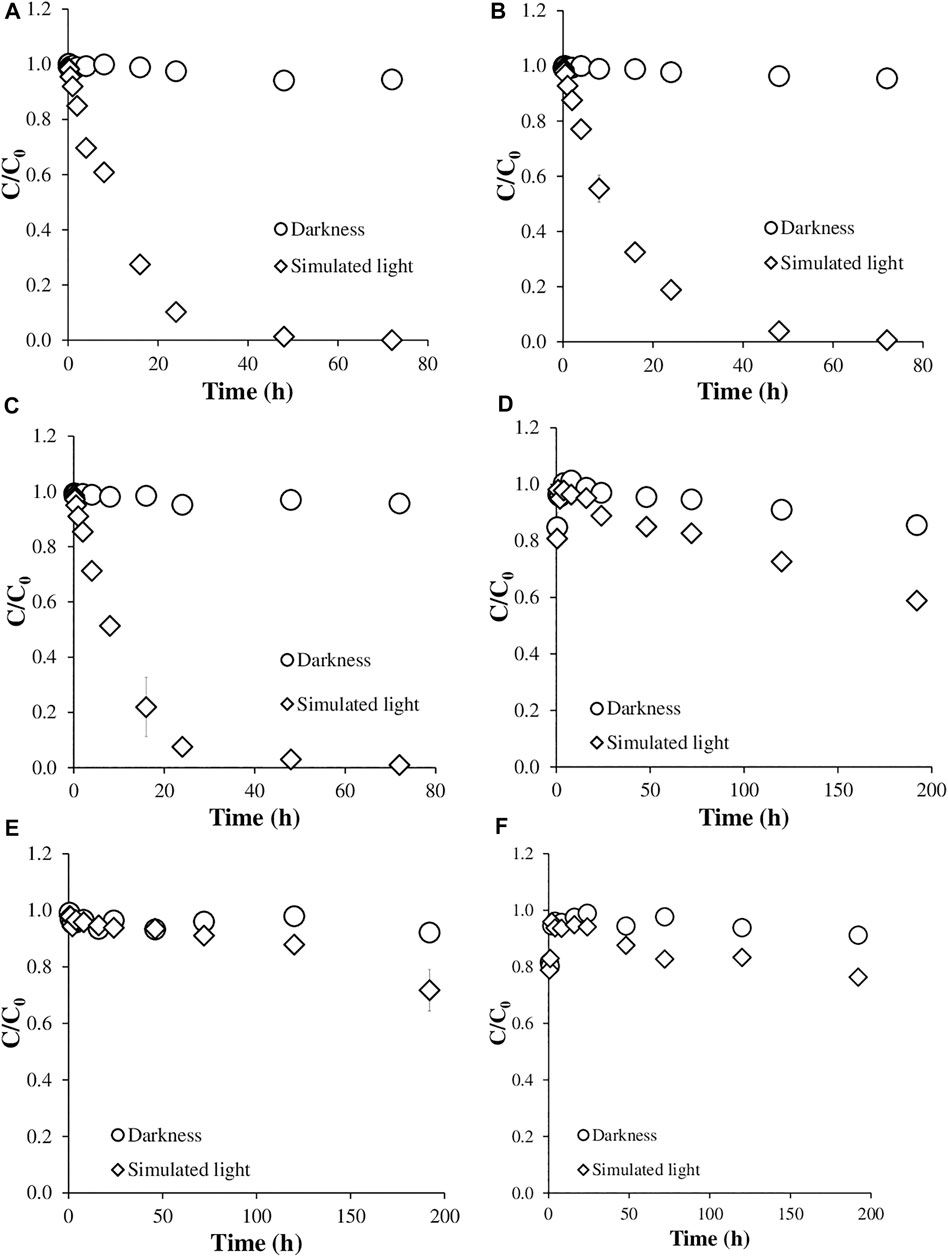
FIGURE 1. Degradation kinetics of the antibiotics studied. (A) Amoxicillin at pH 4.0; (B) Amoxicillin at pH 5.5; (C) Amoxicillin at pH 7.0; (D) Azithromycin at pH 4.0; (E) Azithromycin at pH 5.5; (F) Azithromycin at pH 7.0.
The behavior of AZT in dark conditions was similar to that of AMX, keeping the C/C0 values close to 1 for all the contact times considered (Figures 1D–F). However, the degradation under simulated sunlight was much lower than that of AMX, in spite of using longer times of exposure to light (Figures 1D–F), with C/C0 values always above 0.5. In this case, the degradation was higher at pH 4.0 than at pH 5.5 and 7.2 (Figures 1D–F).
The exponential decay model used to calculate half-lives (Eq. 1) proved to be useful for this type of estimation, with R2 scoring between 0.913 and 0.999 (Table 2). For AMX, the kinetic constants (k) did not show clear variation when pH went from 4.0 to 7.2, with just a slight increase at pH 7.2, with the half-life following the same trend, going from 8.8 h (at pH 4.0) to 7.7 h (at pH 7.2), and reaching a value of 9.9 h at the intermediate pH of 5.5 (Table 2).
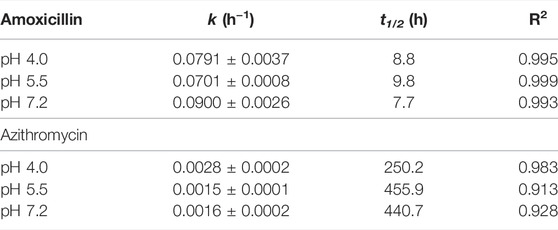
TABLE 2. Data corresponding to the exponential decay model showing the behavior of the antibiotics in filtered milliQ water at different pH values; k: rate constant (h−1); t1/2: half-life (h).
Furthermore, changing the pH between 4 and 7.2 does not produce significant modifications in the AMX adsorption spectra (Figure 2), an aspect that could also influence its degradation, but it does not seem to be relevant in this case.
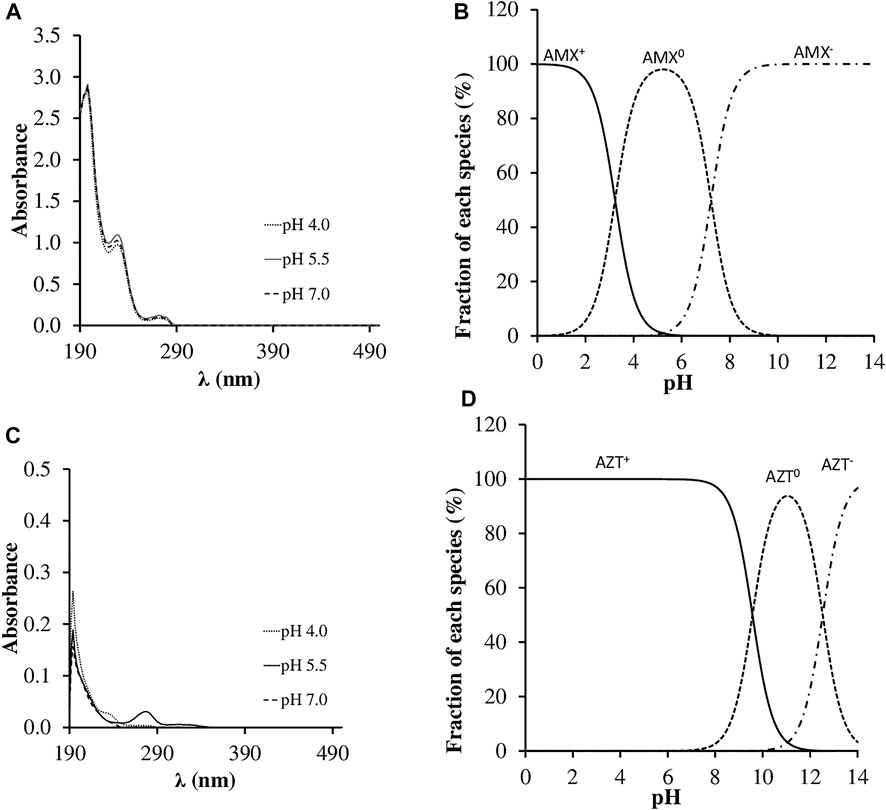
FIGURE 2. Spectra of antibiotics adsorption and speciation at different pH values (4.0, 5.5 and 7.2). (A) Spectrum of Amoxicillin (AMX); (B) Speciation of AMX; (C) Spectrum of Azithromycin (AZT); (D) Speciation of AZT.
Figure 3 presents TOC data corresponding to the experiments with AMX under simulated sunlight at different pHs and at different times of exposition to light. With 2 and 16 h of exposure, TOC does not decrease significantly, despite the fact that the concentration of AMX does reduce by more than 50% at the three pH values studied (Figures 1A–C). After 72 h of simulated sunlight, AMX was below the quantification limit, but the TOC measurements only decreased between 30 and 35%, without significant differences among the three pH values studied (Figure 3).
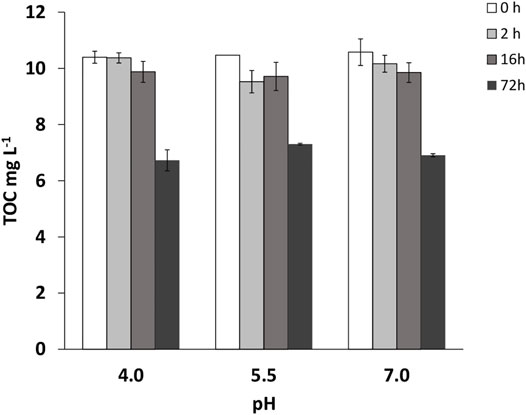
FIGURE 3. Total organic carbon (TOC) values (mg L−1) for Amoxicillin (AMX), at times: 0, 2, 16, and 72 h, in the experiments under simulated sunlight. Average values for three replicates (n = 3), with vertical bars corresponding to standard deviations.
The degradation of AZT was different from that of AMX, with much lower kinetic constants (ranging between 0.0016 and 0.0028 h−1) and much longer half-lives (between 250 and 456 h), the lowest values corresponding to pH 4.0. This indicates that AZT suffers higher degradation at acidic pH (4.0) (Table 2), with a minimum degradation at pH 5.5, which corresponds to a longer half-life (Table 2).
Effects of Inorganic Salts and Humic Acids on AMX and AZT Photo-Degradation
The effects of the presence of the inorganic salts NaCl, CaCl2, NaNO3, Na2HPO4, and FeCl3, and of soluble organic matter (humic acids) on the degradation of the two antibiotics studied, both in the dark and under simulated sunlight, are presented in Figures 4, 5.
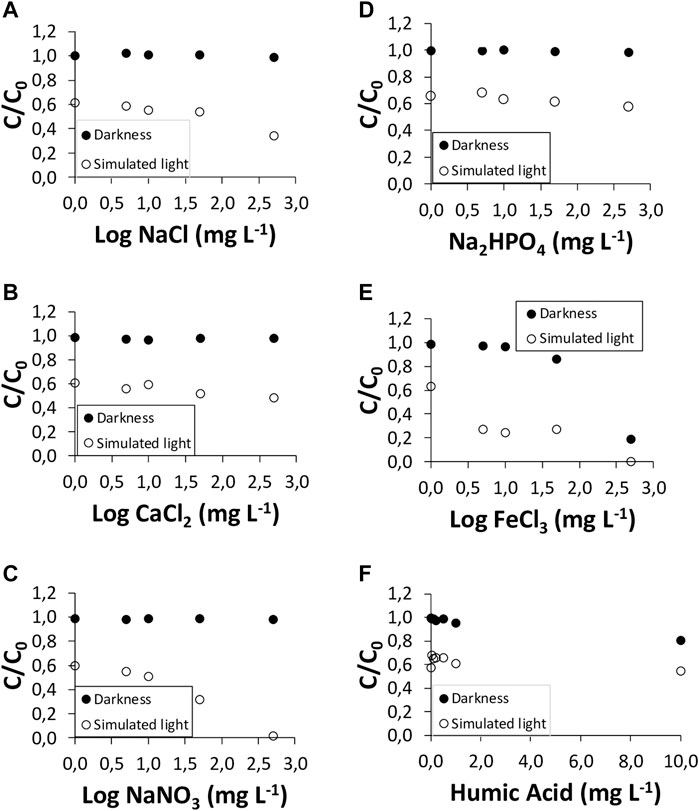
FIGURE 4. Amoxicillin (AMX) degradation under simulated sunlight and in the dark, in the presence of the inorganic salts: (A) NaCl; (B) CaCl2; (C) NaNO3; (D) Na2HPO4; (E) FeCl3; and in the presence of (F) humic acids.
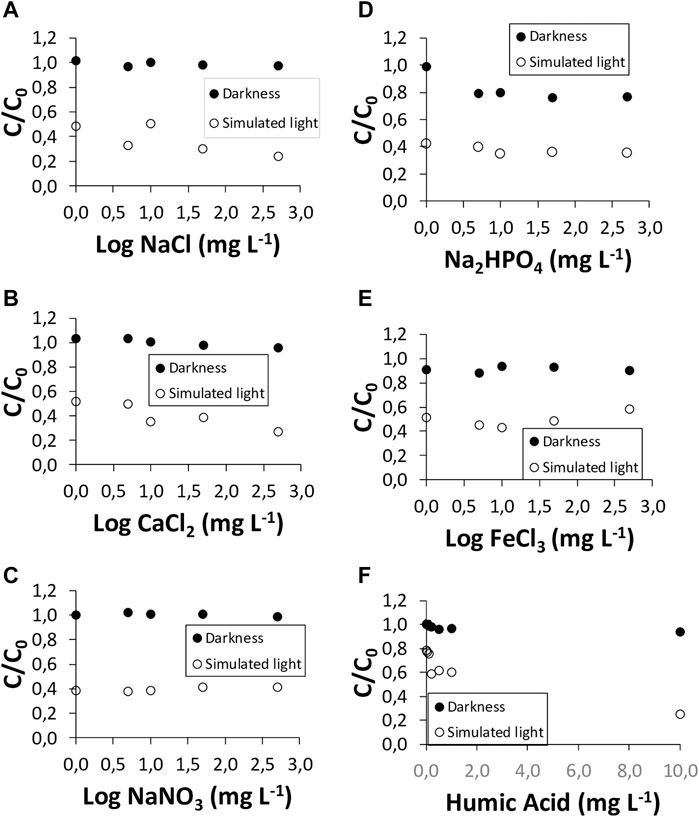
FIGURE 5. Azithromycin (AZT) degradation under simulated sunlight and in the dark, in the presence of the inorganic salts: (A) NaCl; (B) CaCl2; (C) NaNO3; (D) Na2HPO4; (E) FeCl3; and in the presence of (F) humic acids.
Figure 4 shows the data corresponding to AMX. The results indicate that the degradation of AMX is dependent on exposition to light, as well as on the matrix, and on the characteristics of the antibiotic. In the dark, no effect on the degradation of AMX was found for the different inorganic salts studied, except for FeCl3 at its highest concentration (500 mg L−1), which causes a 100% dissipation of AMX; however, under simulated sunlight, AMX degradation was greatly increased in the presence of NaCl and NaNO3 (Figures 1A,C). The inorganic salt NaCl had an effect favoring the dissipation of AMX, with C/C0 passing from a value of 0.61 in the sample without NaCl to 0.34 in the presence of 500 mg L−1 of NaCl, which means a decrease of 44% for this antibiotic (Figure 4A). The presence of NaNO3 caused the dissipation of practically 100% of AMX for the highest concentration of the salt used (500 mg L−1) (Figure 4C).
The effects of CaCl2 and Na2HPO4 under simulated sunlight were slightly lower, with C/C0 values changing from 0.60 to 0.48, and from 0.65 to 0.57, respectively, which represents 21% and 12% dissipation, respectively (Figures 4B,D). As was the case in the dark, the presence of FeCl3 made AMX degradation very high (Figure 1E).
The effect of the presence of inorganic salts on the dissipation of AZT is shown in Figure 5. In the dark, no significant effect was observed, while under simulated sunlight the salts that had the greatest effect on increasing the degradation of AZT were NaCl, (with C/C0 changing from 0.49 to 0.24, which means a dissipation of 51% for the antibiotic) and CaCl2 (with C/C0 changing from 0.52 to 0.27, which means 47% of dissipation) (Figures 5A,B); NaNO3, Na2HPO4 and FeCl3 did not cause increases in AZT degradation (Figures 5C–E). The fundamental difference of AZT with respect to AMX is the lack of effect of FeCl3, and the effect of NaCl and NaNO3 favoring the degradation for AZT, with respect to AMX (Figures 4, 5).
Finally, the presence of dissolved organic matter (humic acids) had very scarce effect on AMX degradation, both in the dark and under simulated sunlight (Figure 4F). There is a slight dissipation taking place both in the dark and under simulated sunlight (4%–19% of increase in dissipation) that can be related to the adsorption of AMX onto humic acids. However, for AZT the results are similar to those of AMX in the dark (6% of increase in dissipation), whereas under simulated sunlight the dissipation is very intense, reaching 68% (Figure 5F).
Discussion
Effect of pH on AMX and AZT Photo-Degradation
Regarding previous publications, there are few data on the photo-degradation of AMX in aqueous solutions without the intervention of a photo-catalyst, but the trend is that the degradation increases as the pH rises, as has been pointed out in studies using TiO2 nanoparticles (Moosavi and Tavakoli, 2016; Li et al., 2019), or ZnO (Elmolla and Chaudhuri, 2010). However, there are also other studies indicating that changing pH does not affect AMX degradation, such as that of Dimitrakopoulou et al. (2012), who reported that the change in pH from 5 to 7.5 had not effect on AMX degradation, but it did slow down the mineralization of the antibiotic. This increase in degradation as the pH rises is usually related to the different sensitivity of the various species present, which is dependent on pH. Specifically, the zwitterion and negatively charged forms grow as the pH increases (Figure 2), which is determined by their pKa values. The effect of increasing photo-degradation with rising pH has also been highlighted for other antibiotics, such as tetracyclines (Conde-Cid et al., 2018a), sulfonamides (Canonica et al., 2008; Conde-Cid et al., 2018b) and other families of antibiotics (Canonica et al., 2008; Rodríguez-López et al., 2021; Álvarez-Esmorís et al., 2022).
Regarding TOC analyses, the results obtained indicate that the mineralization of AMX is not complete and that intermediate molecules are formed through different degradation pathways, such as hydroxylation, hydrolysis and decarboxylation (Li et al., 2019; Zhang et al., 2019).
The behavior of presenting a maximum stability at pH between 5.0 and 6.5, has already been described for AZT by other authors (Farghaly and Mohamed, 2004; Zhang et al., 2009). In addition, Čizmič et al. (2019) described a lack of effect on the degradation of AZT due to varying pH between 3.0 and 7.0, but they did observe an increase in the degradation of AZT when pH reached 10. The greater or minor degree of photo-degradation in this case is not related to different ionic forms of the molecule, since AZT has a predominantly positive charge in the pH range studied in the current work, due to its very high pKa values (Table 1; Figure 2D). Although it was not possible to verify this fact with TOC measurements, numerous intermediate degradation products have also been detected for AZT, generating different fragments due to modifications in the macrolide ring (Tong et al., 2011).
Effects of Salts and Humic Acids on Photo-Degradation
The effect of Fe favoring the degradation of AMX has already been described by several authors, both through the Fenton reagent (Ay and Kargi, 2010) and through Fe particles (Ghauch et al., 2009), the fundamental mechanism being the formation of OH radicals that favor the degradation of the antibiotic (Buitrago et al., 2020).
Comparing with previous publications, the results regarding the effects of different water matrices on the degradation of the antibiotics are contradictory, which can be attributed to the different experimental conditions and the difference in sensitivity of the antibiotics. In this sense, Canonica et al. (2008) indicate that the effects of photosensitizers on the degradation of antibiotics depended on the type of antibiotic and the light source. Different results are also described even within the same family of antibiotics, as is the case of the various responses due to the presence of dissolved organic matter and nitrates on the degradation of different cephalosporin molecules (Jiang et al., 2010). In general, the presence of nitrates in water has been related to an increase in the photolysis of AZT (Tang et al., 2019). In addition, an increase in ionic strength was related to a higher rate of degradation of AZT (Zhang et al., 2009). Other authors found an inhibitory effect due to the presence of anions such as Cl−, HCO3−, NO3−, and SO42-, which has been observed for AMX (Zhang et al., 2019), while some other authors did not find neither inhibition nor promotion of degradation (Mavronikola et al., 2009).
Regarding the effect of humic acids on the dissipation of antibiotics, it probably depends on the content of these organic substances, since at low concentrations they can work as promoters of the photosensitivity of antibiotics, while at high concentrations they can act as a preventer of photo-degradation by increasing the turbidity of the liquid medium (Thiele-Bruhn, 2003; Xuan et al., 2010). This is one of the reasons why contradictory results are found regarding its effect on the degradation of antibiotic molecules. As example, an effect of increasing the dissipation of sulfonamides has been previously reported (Conde-Cid et al., 2018b), but their presence caused no effect on the dissipation of tetracycline (Chen et al., 2008).
Conclusion
Among the main facts to remark from the current study, it should be noted that the antibiotics AMX and AZT did not show significant dissipation in the dark. AMX presented a half-life under simulated sunlight that ranged between 7.7 and 8.7 h, with the lowest value associated to the highest pH in the range investigated (pH 7.2). On the other hand, AZT presented a higher half-life, with values that ranged between 250 and 456 h, showing the highest value for an intermediate pH (5.5). AMX completely dissipated after 50 h of simulated sunlight, but the evaluation of the TOC values indicates that the mineralization ranged between 30% and 35%, which corresponds to the formation of intermediate compounds. However, AZT did not completely dissipate even for the longest contact time used (216 h) for the three pH values tested (4.0, 5.5, and 7.2). The presence of NaCl had a favorable effect on the dissipation of both antibiotics, with an increase in degradation of 44% and 51% for AMX and AZT, respectively. The presence of CaCl2 increased the dissipation of AMX by 21%, and 47% for AZT. NaNO3 caused the total dissipation of AMX when this salt was present at high concentrations, while it had no effect on AZT. The presence of Na2HPO4 had no effect on the dissipation of the two antibiotics studied, while FeCl3 drastically increased AMX dissipation both in the dark and under simulated sunlight, and had no effect on AZT. The presence of humic acids only slightly increased the dissipation of AMX, both in the dark (17%) and under simulated sunlight (4%), having a similar effect for AZT in the dark (6% increase in dissipation), and being more pronounced under simulated sunlight, with an increase in dissipation of 68% for the highest concentration of these substances (10 mg L−1). These results can be considered relevant to understand and predict the fate of both antibiotics when they reach environmental compartments, and could help to define appropriate conditions to achieve the inactivation/degradation/removal of these emerging pollutants.
Data Availability Statement
The raw data supporting the conclusion of this article will be made available by the authors, without undue reservation.
Author Contributions
All authors listed have made a substantial, direct, and intellectual contribution to the work and approved it for publication.
Funding
This work was supported by the Spanish Ministry of Economy and Competitiveness (grant numbers RTI2018-099574-B-C21 and RTI2018-099574-B-C22), with European Regional Development Funds (FEDER in Spain). LR-L holds a predoctoral FPU contract (FPU19/03758 Ministry of Education and Professional Formation) and PP-R is supported by a Postdoctoral fellowship from Xunta de Galicia (ED481D-2021/016).
Conflict of Interest
The authors declare that the research was conducted in the absence of any commercial or financial relationships that could be construed as a potential conflict of interest.
References
Almansba, A., Kane, A., Nasrallah, N., Wilson, J. M., Maachi, R., Lamaa, L., et al. (2021). An Engineering Approach Towards the Design of an Innovative Compact Photo-Reactor for Antibiotic Removal in the Frame of Laboratory and Pilot-Plant Scale. J. Photochem. Photobiol. A Chem. 418, 113445. doi:10.1016/j.jphotochem.2021.113445
Álvarez-Esmorís, C., Rodríguez-López, L., Fernández-Calviño, D., Núñez-Delgado, A., Álvarez-Rodríguez, E., and Arias-Estévez, M. (2022). Degradation of Doxycycline, Enrofloxacin, and Sulfamethoxypyridazine Under Simulated Sunlight at Different pH Values and Chemical Environments. Agronomy 12, 260. doi:10.3390/agronomy12020260
Arun, S., Kumar, R. M., Ruppa, J., Mukhopadhyay, M., Ilango, K., and Chakraborty, P. (2020). Occurrence, Sources and Risk Assessment of Fluoroquinolones in Dumpsite Soil and Sewage Sludge from Chennai, India. Environ. Toxicol. Pharmacol. 79, 103410. doi:10.1016/j.etap.2020.103410
Ay, F., and Kargi, F. (2010). Advanced Oxidation of Amoxicillin by Fenton's Reagent Treatment. J. Hazard. Mater. 179, 622–627. doi:10.1016/j.jhazmat.2010.03.048
Bavumiragira, J. P., Ge, J. n., and Yin, H. (2022). Fate and Transport of Pharmaceuticals in Water Systems: A Processes Review. Sci. Total Environ. 823, 153635. doi:10.1016/j.scitotenv.2022.153635
Bilal, M., Mehmood, S., Rasheed, T., and Iqbal, H. M. N. (2020). Antibiotics Traces in the Aquatic Environment: Persistence and Adverse Environmental Impact. Curr. Opin. Environ. Sci. Health 13, 68–74. doi:10.1016/j.coesh.2019.11.005
Brandt, K. K., Amézquita, A., Backhaus, T., Boxall, A., Coors, A., Heberer, T., et al. (2015). Ecotoxicological Assessment of Antibiotics: A Call for Improved Consideration of Microorganisms. Environ. Int. 85, 189–205. doi:10.1016/j.envint.2015.09.013
Buitrago, J. L., Sanabria, J., Gútierrez-Zapata, H. M., Urbano-Ceron, F. J., García-Barco, A., Osorio-Vargas, P., et al. (2020). Photo-Fenton Process at Natural Conditions of pH, Iron, Ions, and Humic Acids for Degradation of Diuron and Amoxicillin. Environ. Sci. Pollut. Res. 27, 1608–1624. doi:10.1007/s11356-019-06700-y
Canonica, S., Meunier, L., and von Gunten, U. (2008). Phototransformation of Selected Pharmaceuticals During UV Treatment of Drinking Water. Water Res. 42, 121–128. doi:10.1016/j.watres.2007.07.026
Chaturvedi, P., Giri, B. S., Shukla, P., and Gupta, P. (2021). Recent Advancement in Remediation of Synthetic Organic Antibiotics from Environmental Matrices: Challenges and Perspective. Bioresour. Technol. 319, 124161. doi:10.1016/j.biortech.2020.124161
Chen, Y., Hu, C., Qu, J., and Yang, M. (2008). Photodegradation of Tetracycline and Formation of Reactive Oxygen Species in Aqueous Tetracycline Solution under Simulated Sunlight Irradiation. J. Photochem. Photobiol. A Chem. 197, 81–87. doi:10.1016/j.jphotochem.2007.12.007
Čizmić, M., Ljubas, D., Rožman, M., Ašperger, D., Ćurković, L., and Babić, S. (2019). Photocatalytic Degradation of Azithromycin by Nanostructured TiO2 Film: Kinetics, Degradation Products, and Toxicity. Materials 12, 873. doi:10.3390/ma12060873
Conde-Cid, M., Fernández-Calviño, D., Nóvoa-Muñoz, J. C., Arias-Estévez, M., Díaz-Raviña, M., Fernández-Sanjurjo, M. J., et al. (2018a). Biotic and Abiotic Dissipation of Tetracyclines Using Simulated Sunlight and in the Dark. Sci. Total Environ. 635, 1520–1529. doi:10.1016/j.scitotenv.2018.04.233
Conde-Cid, M., Fernández-Calviño, D., Nóvoa-Muñoz, J. C., Arias-Estévez, M., Díaz-Raviña, M., Núñez-Delgado, A., et al. (2018b). Degradation of Sulfadiazine, Sulfachloropyridazine and Sulfamethazine in Aqueous Media. J. Environ. Manag. 228, 239–248. doi:10.1016/j.jenvman.2018.09.025
Cycoń, M., Mrozik, A., and Piotrowska-Seget, Z. (2019). Antibiotics in the Soil Environment-Degradation and Their Impact on Microbial Activity and Diversity. Front. Microbiol. 10, 338. doi:10.3389/fmicb.2019.00338
Dimitrakopoulou, D., Rethemiotaki, I., Frontistis, Z., Xekoukoulotakis, N. P., Venieri, D., and Mantzavinos, D. (2012). Degradation, Mineralization and Antibiotic Inactivation of Amoxicillin by UV-A/TiO2 Photocatalysis. J. Environ. Manag. 98, 168–174. doi:10.1016/j.jenvman.2012.01.010
Elmolla, E. S., and Chaudhuri, M. (2010). Degradation of Amoxicillin, Ampicillin and Cloxacillin Antibiotics in Aqueous Solution by the UV/ZnO Photocatalytic Process. J. Hazard. Mater. 173, 445–449. doi:10.1016/j.jhazmat.2009.08.104
Farghaly, O. A. E.-M., and Mohamed, N. A. L. (2004). Voltammetric Determination of Azithromycin at the Carbon Paste Electrode. Talanta 62, 531–538. doi:10.1016/j.talanta.2003.08.026
Forsberg, K. J., Reyes, A., Wang, B., Selleck, E. M., Sommer, M. O. A., and Dantas, G. (2012). The Shared Antibiotic Resistome of Soil Bacteria and Human Pathogens. Science 337, 1107–1111. doi:10.1126/science.1220761
Ghauch, A., Tuqan, A., and Assi, H. A. (2009). Antibiotic Removal from Water: Elimination of Amoxicillin and Ampicillin by Microscale and Nanoscale Iron Particles. Environ. Pollut. 157, 1626–1635. doi:10.1016/j.envpol.2008.12.024
Ghirardini, A., Grillini, V., and Verlicchi, P. (2020). A Review of the Occurrence of Selected Micropollutants and Microorganisms in Different Raw and Treated Manure - Environmental Risk Due to Antibiotics after Application to Soil. Sci. Total Environ. 707, 136118. doi:10.1016/j.scitotenv.2019.136118
Han, N., Yao, Z., Ye, H., Zhang, C., Liang, P., Sun, H., et al. (2019). Efficient Removal of Organic Pollutants by Ceramic Hollow Fibre Supported Composite Catalyst. Sustain. Mater. Technol. 20, e00108. doi:10.1016/j.susmat.2019.e00108
Han, N., Race, M., Zhang, W., Marotta, R., ZhangBokhari, C. A., Bokhari, A., et al. (2021). Perovskite and Related Oxide Based Electrodes for Water Splitting. J. Clean. Prod. 318, 128544. doi:10.1016/j.jclepro.2021.128544
Hassan, M. M., El Zowalaty, M. E., Lundkvist, Å., Järhult, J. D., Khan Nayem, M. R., Tanzin, A. Z., et al. (2021). Residual Antimicrobial Agents in Food Originating from Animals. Trends Food Sci. Technol. 111, 141–150. doi:10.1016/j.tifs.2021.01.075
Iwu, C. D., Korsten, L., and Okoh, A. I. (2020). The Incidence of Antibiotic Resistance within and beyond the Agricultural Ecosystem: A Concern for Public Health. MicrobiologyOpen 9, e1035. doi:10.1002/mbo3.1035
Jiang, M., Wang, L., and Ji, R. (2010). Biotic and Abiotic Degradation of Four Cephalosporin Antibiotics in a Lake Surface Water and Sediment. Chemosphere 80 (11), 1399–1405. doi:10.1016/j.chemosphere.2010.05.048
Kemper, N. (2008). Veterinary Antibiotics in the Aquatic and Terrestrial Environment. Ecol. Indic. 8, 1–13. doi:10.1016/j.ecolind.2007.06.002
Khan, H. K., Rehman, M. Y. A., and Malik, R. N. (2020). Fate and Toxicity of Pharmaceuticals in Water Environment: An Insight on Their Occurrence in South Asia. J. Environ. Manag. 271, 111030. doi:10.1016/j.jenvman.2020.111030
Kumar, S. B., Arnipalli, S. R., and Ziouzenkova, O. (2020). Antibiotics in Food Chain: The Consequences for Antibiotic Resistance. Antibiotics 9, 688. doi:10.3390/antibiotics9100688
Li, Q., Jia, R., Shao, J., and He, Y. (2019). Photocatalytic Degradation of Amoxicillin via TiO2 Nanoparticle Coupling with a Novel Submerged Porous Ceramic Membrane Reactor. J. Clean. Prod. 209, 755–761. doi:10.1016/j.jclepro.2018.10.183
Liu, X., Zhou, Y., Zhang, J., Luo, L., Yang, Y., Huang, H., et al. (2018). Insight into Electro-Fenton and Photo-Fenton for the Degradation of Antibiotics: Mechanism Study and Research Gaps. Chem. Eng. J. 347, 379–397. doi:10.1016/j.cej.2018.04.142
Manyi-Loh, C., Mamphweli, S., Meyer, E., and Okoh, A. (2018). Antibiotic Use in Agriculture and its Consequential Resistance in Environmental Sources: Potential Public Health Implications. Molecules 23, 795. doi:10.3390/molecules23040795
Mavronikola, C., Demetriou, M., Hapeshi, E., Partassides, D., Michael, C., Mantzavinos, D., et al. (2009). Mineralisation of the Antibiotic Amoxicillin in Pure and Surface Waters by Artificial UVA- and Sunlight-Induced Fenton Oxidation. J. Chem. Technol. Biotechnol. 84, 1211–1217. doi:10.1002/jctb.2159
Moosavi, F. S., and Tavakoli, T. (2016). Amoxicillin Degradation from Contaminated Water by Solar Photocatalysis Using Response Surface Methodology (RSM). Environ. Sci. Pollut. Res. 23, 23262–23270. doi:10.1007/s11356-016-7349-y
Muhammad, J., Khan, S., Su, J. Q., Hesham, A. E.-L., Ditta, A., Nawab, J., et al. (2020). Antibiotics in Poultry Manure and Their Associated Health Issues: A Systematic Review. J. Soils Sediments 20, 486–497. doi:10.1007/s11368-019-02360-0
Nippes, R. P., Macruz, P. D., da Silva, G. N., and Neves Olsen Scaliante, M. H. (2021). A Critical Review on Environmental Presence of Pharmaceutical Drugs Tested for the Covid-19 Treatment. Process Saf. Environ. Prot. 152, 568–582. doi:10.1016/j.psep.2021.06.040
Nnadozie, C. F., and Odume, O. N. (2019). Freshwater Environments as Reservoirs of Antibiotic Resistant Bacteria and Their Role in the Dissemination of Antibiotic Resistance Genes. Environ. Pollut. 254 (B), 113067. doi:10.1016/j.envpol.2019.113067
Rico, A., Vighi, M., Van den Brink, P. J., Horst, M., Macken, A., Lillicrap, A., et al. (2019). Use of Models for the Environmental Risk Assessment of Veterinary Medicines in European Aquaculture: Current Situation and Future Perspectives. Rev. Aquacult. 11, 969–988. doi:10.1111/raq.12274
Rodríguez-López, L., Cela-Dablanca, R., Núñez-Delgado, A., Álvarez-Rodríguez, E., Fernández-Calviño, D., and Arias-Estévez, M. (2021). Photodegradation of Ciprofloxacin, Clarithromycin and Trimethoprin: Influence of pH and Humic Acids. Molecules 26, 3080. doi:10.3390/molecules.26113080
Salam, L. B., and Obayori, O. S. (2019). Structural and Functional Metagenomic Analyses of a Tropical Agricultural Soil. Span. J. Soil Sci. 9 (1), 1–23. doi:10.3232/SJSS.2019.V9.N1.01
Santás-Miguel, V., Díaz-Raviña, M., Martín, A., García-Campos, E., Barreiro, A., Núñez-Delgado, A., et al. (2020). Medium-Term Influence of Tetracyclines on Total and Specific Microbial Biomass in Cultivated Soils of Galicia (NW Spain). Span. J. Soil Sci. 10, 217–232. doi:10.3232/SJSS.2020.V10.N3.05
Serwecińska, L. (2020). Antimicrobials and Antibiotic-Resistant Bacteria: A Risk to the Environment and to Public Health. Water 12, 3313. doi:10.3390/w12123313
Silva, C., Louros, V., Silva, V., Otero, M., and Lima, D. (2021). Antibiotics in Aquaculture Wastewater: Is it Feasible to Use a Photodegradation-Based Treatment for Their Removal? Toxics 9, 194. doi:10.3390/toxics9080194
Sodhi, K. K., Kumar, M., and Singh, D. K. (2021). Insight into the Amoxicillin Resistance, Ecotoxicity, and Remediation Strategies. J. Water Process Eng. 39, 101858. doi:10.1016/j.jwpe.2020.101858
Solliec, M., Roy-Lachapelle, A., Gasser, M.-O., Coté, C., Généreux, M., and Sauvé, S. (2016). Fractionation and Analysis of Veterinary Antibiotics and Their Related Degradation Products in Agricultural Soils and Drainage Waters Following Swine Manure Amendment. Sci. Total Environ. 543, 524–535. doi:10.1016/j.scitotenv.2015.11.061
Su, C., Zhang, H., Cridge, C., and Liang, R. (2019). A Review of Multimedia Transport and Fate Models for Chemicals: Principles, Features and Applicability. Sci. Total Environ. 668, 881–892. doi:10.1016/j.scitotenv.2019.02.456
Talaiekhozani, A., Joudaki, S., Banisharif, F., Eskandari, Z., Cho, J., Moghadam, G., et al. (2020). Comparison of Azithromycin Removal from Water Using UV Radiation, Fe (VI) Oxidation Process and ZnO Nanoparticles. Int. J. Environ. Res. Pub. Heal. 17, 1758. doi:10.3390/ijerph17051758
Tang, J., Wang, S., Fan, J., Long, S., Wang, L., Tang, C., et al. (2019). Predicting Distribution Coefficients for Antibiotics in a River Water-Sediment Using Quantitative Models Based on Their Spatiotemporal Variations. Sci. Total Environ. 655, 1301–1310. doi:10.1016/j.scitotenv.2018.11.163
Tello, A., Austin, B., and Telfer, T. C. (2012). Selective Pressure of Antibiotic Pollution on Bacteria of Importance to Public Health. Environ. Health Perspect. 120, 1100–1106. doi:10.1289/ehp.1104650
Thiele-Bruhn, S. (2003). Pharmaceutical Antibiotic Compounds in Soils - A Review. J. Plant Nutr. Soil Sci. 166, 145–167. doi:10.1002/jpln.200390023
Tong, L., Eichhorn, P., Pérez, S., Wang, Y., and Barceló, D. (2011). Photodegradation of Azithromycin in Various Aqueous Systems under Simulated and Natural Solar Radiation: Kinetics and Identification of Photoproducts. Chemosphere 83, 340–348. doi:10.1016/j.chemosphere.2010.12.025
Tran, N. H., Hoang, L., Nghiem, L. D., Nguyen, N. M. H., Ngo, H. H., Guo, W., et al. (2019). Occurrence and Risk Assessment of Multiple Classes of Antibiotics in Urban Canals and Lakes in Hanoi, Vietnam. Sci. Total Environ. 692, 157–174. doi:10.1016/j.scitotenv.2019.07.092
Wang, J., and Wang, S. (2016). Removal of Pharmaceuticals and Personal Care Products (PPCPs) from Wastewater: A Review. J. Environ. Manag. 182, 620–640. doi:10.1016/j.jenvman.2016.07.049
Xuan, R., Arisi, L., Wang, Q., Yates, S. R., and Biswas, K. C. (2010). Hydrolysis and Photolysis of Oxytetracycline in Aqueous Solution. J. Environ. Sci. Health, Part B 45, 73–81. doi:10.1080/03601230903404556
Yang, C.-W., Hsiao, W.-C., and Chang, B.-V. (2016). Biodegradation of Sulfonamide Antibiotics in Sludge. Chemosphere 150, 559–565. doi:10.1016/j.chemosphere.2016.02.064
Yang, Q., Gao, Y., Ke, J., Show, P. L., Ge, Y., Liu, Y., et al. (2021). Antibiotics: An Overview on the Environmental Occurrence, Toxicity, Degradation, and Removal Methods. Bioengineered 12, 7376–7416. doi:10.1080/21655979.2021.1974657
Yi, X., Lin, C., Ong, E. J. L., Wang, M., and Zhou, Z. (2019). Occurrence and Distribution of Trace Levels of Antibiotics in Surface Waters and Soils Driven by Non-point Source Pollution and Anthropogenic Pressure. Chemosphere 216, 213–223. doi:10.1016/j.chemosphere.2018.10.087
Zhang, Y., Liu, X., Cui, Y., Huang, H., Chi, N., and Tang, X. (2009). Aspects of Degradation Kinetics of Azithromycin in Aqueous Solution. Chroma 70, 67–73. doi:10.1365/s10337-009-1116-x
Zhang, Y., Xiao, Y., Zhong, Y., and Lim, T.-T. (2019). Comparison of Amoxicillin Photodegradation in the UV/H2O2 and UV/Persulfate Systems: Reaction Kinetics, Degradation Pathways, and Antibacterial Activity. Chem. Eng. J. 372, 420–428. doi:10.1016/j.cej.2019.04.160
Keywords: amoxicillin, azithromycin, humic acids, inorganic salts, photo-degradation
Citation: Rodríguez-López L, Santás-Miguel V, Núñez-Delgado A, Álvarez-Rodríguez E, Pérez-Rodríguez P and Arias-Estévez M (2022) Influence of pH, Humic Acids, and Salts on the Dissipation of Amoxicillin and Azithromycin Under Simulated Sunlight. Span. J. Soil Sci. 12:10438. doi: 10.3389/sjss.2022.10438
Received: 16 February 2022; Accepted: 26 April 2022;
Published: 25 May 2022.
Edited by:
Xose Luis Otero, Scientific coordinator of REBUSC (Network of Biological Stations of the University of Santiago de Compostela), SpainCopyright © 2022 Rodríguez-López, Santás-Miguel, Núñez-Delgado, Álvarez-Rodríguez, Pérez-Rodríguez and Arias-Estévez. This is an open-access article distributed under the terms of the Creative Commons Attribution License (CC BY). The use, distribution or reproduction in other forums is permitted, provided the original author(s) and the copyright owner(s) are credited and that the original publication in this journal is cited, in accordance with accepted academic practice. No use, distribution or reproduction is permitted which does not comply with these terms.
*Correspondence: Vanesa Santás-Miguel, dnNhbnRhc0B1dmlnby5lcw==