- 1Department of Soil Science and Agricultural Chemistry, Universidad de Santiago de Compostela, Lugo, Spain
- 2Department of Plant Production and Engineering Projects, Universidad de Santiago de Compostela, Lugo, Spain
- 3Institute of Agricultural Biodiversity and Rural Development, University of Santiago de Compostela, Lugo, Spain
The application of cattle slurry to agricultural soils contributes to the circular economy, while enriching the soil in macro and micronutrients and organic matter. However, this practice can have deleterious environmental effects, by adding toxic metals and other contaminants. The pseudo-total concentrations of nine potentially toxic and trace metals (Fe, Mn, Zn, Cu, Ni, Co, Cr, Cd and Pb) as well as metals extracted by DTPA, Mehlich 3 and 0.01 M CaCl2 were determined in Galician (NW Spain) grassland soils regularly receiving cattle slurry. Four soil depths (0–5, 5–10, 10–20 and 20–40 cm) were sampled and analysed. The pollution condition was assessed by comparing the pseudo-total concentrations with generic reference levels for Galician soils and by using pollution indices. The results indicated the absence of soil pollution by Fe, Ni, Co, Cr and Pb and a situation of no pollution to moderate pollution by Mn, Zn and Cu. Cd was the element most frequently enriched in the studied soils according to the pseudo-total, DTPA and Mehlich-3 concentrations, while the extraction by CaCl2 pointed to no environmental risk. The study supports the lithogenic character of Fe, Ni, Co and Cr, the mixed lithogenic and anthropogenic nature of Mn, Zn and Cu and the anthropogenic origin of Cd in these soils. The latter element can be added by both the application of cattle slurry and inorganic phosphate fertilisers.
Introduction
The term “heavy metals” has been used to refer to metals (sometimes metalloids are included) that in the elemental state have a density greater than 5 g cm−3 and can be toxic to organisms or ecosystems. Many of these elements are present in soils and the lithosphere in tiny concentrations (<0.1%) and are known as “trace elements.” Some trace elements are micronutrients for plants or animals, while others do not have any known biological function. The use of the term “heavy metals” has been questioned, especially in recent years (Nieboer and Richardson, 1980; Duffus, 2002; Hodson, 2004) and the terms “trace elements,” “toxic elements,” “potentially toxic elements,” “soft acid metal ions” or “class B metal ions” are preferred by some authors (Nieboer and Richardson, 1980; Madrid, 2010; Pourret and Hursthouse, 2019; Zhang et al., 2022). However, the term “heavy metals” is often found in current publications and other authors support continuing to use it, as a well-established expression utilised for decades in the environmental field (Batley, 2012; Gustin et al., 2021).
Potentially toxic metals are present in natural soils, usually at trace levels, originating from the parent material. Anthropogenic activities can contribute potentially toxic metals to the soil, promoting the build-up of these elements. Among the anthropogenic sources of potentially toxic metals in soils, mining, metal smelting, burning of fossil fuels, waste disposal and incineration, and traffic of vehicles are worth mentioning. Agricultural activities can also add potentially toxic metals to the soil.
Potentially toxic metals can be found in soil in various chemical forms, those that are soluble or mobile being the most worrying from an environmental point of view in the short/medium term. The soil is often capable of effectively retaining potentially toxic metals and other pollutants, preventing their passage into water bodies and vegetation (Nunez-Delgado et al., 2002). A polluted soil no longer plays a protective role of other environmental compartments, becoming instead a source of pollutants (Richter et al., 1997).
Human being exerts a series of disturbing actions on agricultural soils, among them the addition of fertilisers and pesticides. Various agricultural practices contribute potentially toxic metals to the soil. Many traditionally used fungicides and insecticides supply Cu, Hg, Mn, Pb and Zn (Nicholson et al., 2003). Other agricultural practices contribute potentially toxic metals to the soil from fuels, lubricants, tires and sewage sludge (Nicholson et al., 2003; Antoniadis et al., 2008; Proshad et al., 2021; Li et al., 2022). Manures and slurries from livestock activities may contain significant amounts of Zn, Cu and other heavy metals, which come from additives in animal feed (Mantovi et al., 2003; Jakubus et al., 2013). Zn and Cu are essential elements to plants, but they are toxic at high concentrations. Also chemical fertilisers, in particular phosphates, can contain toxic metals as impurities (Loganathan et al., 2008; Cakmak et al., 2010). Heavy metals can be toxic to soil microorganisms, plants and animals as well as be exported to water bodies and to the food chain (Liang and Tabatabai, 1977; Fernandez-Calvino et al., 2010; Nagajyoti et al., 2010; Antunes et al., 2011; Kabata-Pendias, 2011).
The use of livestock manures and slurries as fertilisers is a sound way of disposal of these animal wastes and allows the use of synthetic fertilisers to be reduced, improving soil properties and crop yields while contributing to a circular economy (Poulsen et al., 2013; Xia et al., 2017). Despite their overall benefit for agriculture, their contribution of potentially toxic metals as well as pathogens and antibiotics residues requires careful management of the application of manure and slurry to agricultural soils. The application of slurry to soil has been reported to be a cause of toxic metal contamination (Del Castilho et al., 1993; Baize, 1997; L'Herroux et al., 1997; Smith, 1997; Romkens and Salomons, 1998; De la Torre et al., 2000; Aldrich et al., 2002; Moreno-Caselles et al., 2002; Wilcke et al., 2002; De Temmerman et al., 2003). The metal toxicity in soils depends not only on their total concentration but, especially, on the concentration of soluble or easily mobilisable forms (McBride, 1989; Alloway and Jackson, 1991; Gupta et al., 1996). In addition, soil contamination can lead to contamination of aquifers as well as contamination of the food chain through vegetation. In relation to the possible contamination of other media, the soil can play a filter role, retaining heavy metals and preventing their passage to groundwater or vegetation (L'Herroux et al., 1997; Nunez-Delgado et al., 2002).
In Galicia (NW Spain), the application of cattle slurry to pastureland is a common practice (Franco-Uria et al., 2009), which provides the soil with macro and micronutrients as well as organic matter. Few published data exist relative to the presence of toxic and trace metals in Galician soils treated with cattle slurry (Paz-González et al., 2000; Caridad-Cancela et al., 2002; Lopez-Mosquera et al., 2005; Franco-Uria et al., 2009; Seco-Reigosa et al., 2015).
Franco-Uría et al. (2009) studied the total concentrations of heavy metals in the surface horizons of grassland soils in the river Magdalena basin (Lugo, Galicia, Spain), which usually receive dairy cattle slurry, with the main aim of identifying the sources of the different heavy metals. The present study addresses the occurrence and quantification of different forms of heavy metals at various soil depths in the same basin, to assess the soil pollution degree, the vertical distribution of metals and the risk of toxic metals mobilisation. Among the studied elements, iron (Fe) is not a trace element in geochemistry (its concentration in the Earth’s crust is 5%), but is a trace element in biochemistry (IUPAC, 2022) and a plant micronutrient.
Materials and Methods
Study Area
The study was carried out in the basin of the river Magdalena, in A Pastoriza, province of Lugo, Spain (43° 16′44″N, 7° 20′50″W). The basin (Figure 1) covers an area of 2,250 ha and is mainly occupied by grasslands (84% of the surface). The principal economic activity in the area is dairy farming. The basin can be considered representative of the zones of intensive cattle breeding in Galicia (NW Spain). The climate is humid temperate, with an annual average temperature of 12°C and average annual precipitation of 1,350 mm (MeteoGalicia, 2022). The soils, developed from tertiary and quaternary sediments and quartz-rich slates, are classified as Umbrisols (IUSS Working Group WRB, 2022). The grassland soils, acid and rich in organic matter (Franco-Uria et al., 2009), are regularly fertilised with cattle slurry, coming from the farms, and inorganic fertilisers. The average contribution of slurry to the soils of the basin is 90 m3 ha−1 year−1. The soil textures range from sandy loam to silt loam and clay loam.
Soil Sampling and Analysis
Twenty farms were selected in the river Magdalena basin, where representative soils were sampled at four depths (0–5, 5–10, 10–20 and 20–40 cm). Each soil sample was made up of 10–15 sub-samples, taken at random from the whole area of each plot with a split-tube sampler. Samples were air-dried and sieved (<2 mm) before analysis. The soil pH in water was 5.42 ± 0.34 at 0–5 cm and 5.28 ± 0.29 at 20–40 cm. The concentration of organic carbon was 7.76% ± 2.01% at 0–5 cm and 3.64% ± 1.20% at 20–40 cm.
Various forms (pseudo-total, DTPA-extractable, Mehlich 3-extractable and 0.01 M CaCl2-extractable) of iron (Fe), manganese (Mn), zinc (Zn), copper (Cu), chromium (Cr), cobalt (Co), nickel (Ni), cadmium (Cd) and lead (Pb) were analysed. All of them are class B or mostly borderline metal ions (Nieboer and Richardson, 1980; Duffus, 2002). Fe, Mn, Zn, Cu and Ni are plant micronutrients. They can all be toxic above a concentration threshold.
Pseudo-total metals were determined by microwave-assisted digestion, with concentrated HNO3 and HCl (HCl:HNO3 ratio 1:3), according to the EPA 3051A method (USEPA, 2007) (Supplementary Data Sheet S2). Available metals were extracted by DTPA (Lindsay and Norvell, 1978) (Supplementary Data Sheet S2). The soil samples were also extracted by Mehlich 3 reagent (Mehlich, 1984) (Supplementary Data Sheet S2) to compare the results obtained with those from DTPA extraction. DTPA and Mehlich 3 reagent are commonly used to determine available trace elements in soils. The Mehlich 3 extraction is less time-consuming than the DTPA extraction and allows the determination of available macronutrients, such as phosphorus, in the same extract. Soluble (mobile) metals were extracted by 0.01M CaCl2 at a soil:solution ratio of 1:10 (Houba et al., 1990). The digestion and extraction methods are described in Supplementary Data Sheet S2. In all cases, the metals were determined in the digestate or extract by ICP-MS. The limits of detection were calculated as 3 times the standard deviation of blank values and are presented in Table 1.
Pollution Assessment
The pseudo-total metal concentrations were compared with the generic reference levels (GRL) for trace elements in Galician soils (Table 2) (Macías-Vázquez and Calvo de Anta, 2009). These levels represent the “concentration of a contaminating substance in the soil that does not carry a risk greater than the maximum acceptable for human or ecosystem health.”
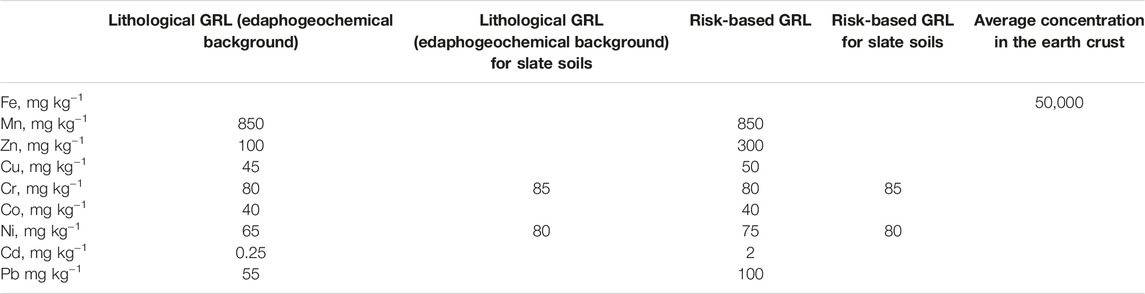
TABLE 2. Generic reference levels (GRL) for various metals in Galician soils (Macías and Calvo de Anta, 2009), including the specific values for soils on slates and the average concentration of iron in the Earth’s crust.
We used two indices to assess pollution in the studied soils. The first one is the enrichment factor (EF) developed by Buat-Menard and Chesselet (1979), which allows to compare a metal concentration in soil with the background concentration, both standardised against a reference element (Al, Fe, or Ti). In the present study Fe was chosen as the reference element, following the recommendations of Daskalakis and Oconnor (1995), Acevedo-Figueroa et al. (2006) and Rodriguez-Seijo et al. (2017), so that EF was calculated as follows:
where X is the metal of interest; EFX, the enrichment factor of the element X; CX, the total concentration of X; CFe, the total concentration of iron (a lithogenic reference element); “soil” refers to the assessed soil, and “background” to the situation of natural unpolluted soils in the study area, taken as a reference. In the present study, the edaphogeochemical background (lithological GRL) values for Galician soils (Table 2) were used as references. EF values below 2 indicate minimum enrichment; values between 2 and 5 represent moderate enrichment; values between 5 and 20 denote significant enrichment; EF values higher than 20 show very high enrichment; values above 40 reveal extremely high enrichment (Rodriguez-Seijo et al., 2017).
The second index used is the geoaccumulation index (Igeo) (Müller, 1979), calculated as:
where CX is the total concentration of the element X and BX is the background concentration of the element X. The factor 1.5 is used to minimise the effect of potential variations in the background value because of lithological variations. We used as background the edaphogeochemical backgrounds for Galician soils (Table 2) and the average concentration in the Earth’s crust for iron. The Igeo values allow to classify the soils into seven categories: <0 = practically unpolluted, 0–1 = unpolluted to moderately polluted, 1–2 = moderately polluted, 2–3 = moderately to strongly polluted, 3–4 = strongly polluted, 4–5 = strongly to extremely polluted, and >5 = extremely polluted (Rodriguez-Seijo et al., 2017).
Statistical Analysis
The analysis of variance was used to assess the influence of depth on the concentrations of the various forms of metals. Correlation analysis was performed between the different forms of each metal. The statistical analysis was performed using the IBM SPSS Statistics 28.0.
Results
Pseudo-Total Metal Concentrations
The concentrations of pseudo-total metals at various depths (means and standard deviations) are presented in Figure 2. Table 2 shows the generic reference levels (GRL) for the studied metals in Galician (NW Spain) soils (Macías-Vázquez and Calvo de Anta, 2009). The specific (exceptional) values for soils on slates are presented when they exist. For iron the average concentration in the Earth’s crust is presented (there is no generic reference level for Fe).
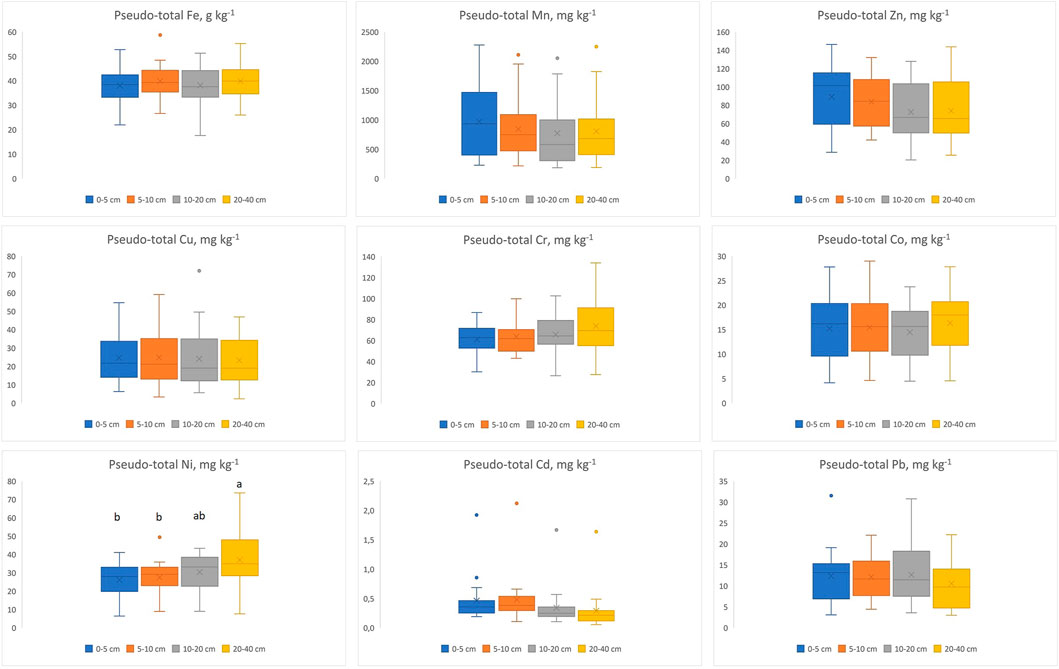
FIGURE 2. Concentrations of pseudo-total metals for different soils as a function of depth. Different letters denote significant differences (p < 0.05) among depths.
The Fe concentrations (Figure 2) ranged between 17.63 and 58.70 g kg−1, being below or very close to its average concentration in the Earth’s crust. The Mn concentrations, ranging from 184 to 2,276 mg kg−1, were above the GRL of 850 mg kg−1 at all depths in seven soils and in the surface (0–5 or 0–10 cm) of four other soils. The Zn concentrations, ranging from 20 to 146 mg kg−1, were above the edaphogeochemical background (100 mg kg−1) with some frequency, but never reached the risk-based GRL (300 mg kg−1). The Cu concentrations, ranging from 2 to 72 mg kg−1, were below the edaphogeochemical background (45 mg kg−1) and the risk-based GRL (50 mg kg−1), excepting two soils. The Cr concentrations ranging from 26 to 134 mg kg−1, exceeded the reference value for slate soils (85 mg kg−1) in various cases, particularly in samples at greater depth. The concentrations of Co (4–29 mg kg−1) and Ni (6–74 mg kg−1) were always below the reference values (40 and 80 mg kg−1, respectively). The Cd concentrations, ranging from 0.05 to 2.12 mg kg−1, were often above the edaphogeochemical background of 0.25 mg kg−1; only one outlier (at 5–10 cm depth) exceeded the risk-based GRL of 2 mg kg−1. The Pb concentrations, ranging from 3 to 32 mg kg−1, were always below the edaphogeochemical background (55 mg kg−1) and the risk-based GRL (100 mg kg−1).
Only for Ni the pseudo-total concentration varied significantly with depth (p < 0.05), being highest in the deepest sampled layer (20–40 cm) and lowest at 0–10 cm (Figure 2). For most metals there was a trend to decrease with depth, although the differences were not significant, or to remain stable (Figure 2). For Ni, Co and Cr there was a trend to increase with depth, the differences being significant only for Ni (Figure 2).
Pollution Indices
The enrichment factors (EF, Table 3) of Co (0.20–1.35), Ni (0.17–1.22) and Pb (0.08–0.88) were always lower than 2, indicating minimum enrichment with respect to the edaphogeochemical background (lithological GRL). The Mn EF values ranged between 0.28 and 5.50. Various soils had Mn EF between 2 and 5, indicating moderate enrichment with respect to the edaphogeochemical background; one sample had EF = 5.50, denoting significant enrichment. The EF values varied in the ranges 0.32–2.46, 0.09–2.37 and 0.37–2.03 for Zn, Cu and Cr, respectively. One to three samples from 10–20 or 20–40 cm depth had Zn, Cu and Cr EF slightly higher than 2, indicating moderate enrichment with respect to the edaphogeochemical background. The highest enrichment factors were found for Cd (0.20–8.70), often showing moderate enrichment (EF from 2 to 5) and significant enrichment (EF from 5 to 20) at all depths in one soil. The enrichment factor (Table 3) showed a trend to decrease with depth for some metals, while for other no visible trend was observed. However, the differences among depths were significant (p < 0.05) only for Ni, whose EF increased with depth, in accordance to the variation of its pseudo-total concentration.
The indices of geoaccumulation (Igeo, Table 4) were always negative (practically unpolluted) for Fe (−2.09 to −0.35), Zn (−2.88 to −0.04), Co (−3.85 to −1.05), Ni (−3.92 to −0.41) and Pb (−4.78 to −1.39). This index was between 0 and 1 (unpolluted to moderately polluted) for Cu (range −4.84 to 0.09) and Cr (range −2.18 to 0.16) in one sample (in the case of Cr coinciding with the sample identified by EF as having moderate enrichment). The Mn Igeo (−2.79 to 0.84) was often between 0 and 1 (unpolluted to moderately polluted), in most cases coinciding with values of EF between 2 and 5 (moderate enrichment). The same as for EF, the highest values of Igeo were found for Cd (−2.82 to 2.50), with frequent values between 0 and 1 (unpolluted to moderately polluted); in one case the value was between 1 and 2 (moderately polluted); Igeo values between 2 and 3 (moderately to strongly polluted) were determined for the soil showing significant enrichment (EF from 5 to 20). The trends of variation of Igeo with depth were similar to those of pseudo-total concentrations and EF. The differences in Igeo values among depths were significant only for Cd, showing the highest values at 0–10 cm (Table 4).
Available and Soluble Metals
Available metal concentrations (DTPA and Mehlich 3) are shown in Figure 3. Metal concentrations determined by DTPA and Mehlich 3 extractions correlated significantly (p < 0.001) for all metals except Cr (Table 5).
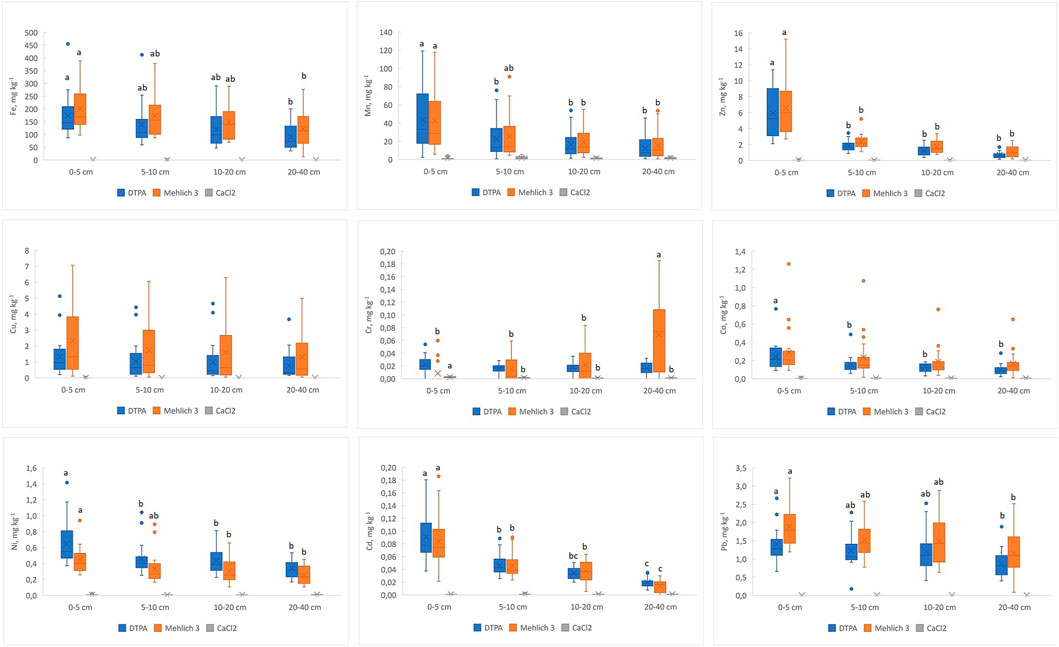
FIGURE 3. Concentrations of available (extractable by DTPA and Mehlich 3) and soluble (extractable by 0.01M CaCl2) metals for different soils as a function of depth. Different letters denote significant differences (p < 0.05) among depths.
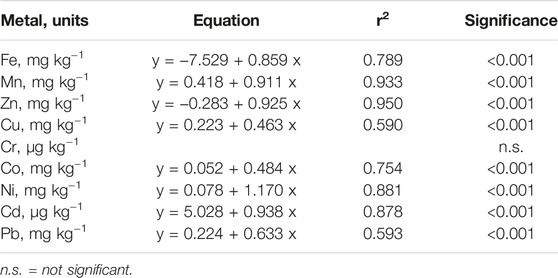
TABLE 5. Regression equations between Mehlich 3 and DTPA extractions. The concentration determined by DTPA extraction is the dependent variable (y) and that determined by Mehlich 3 extraction is the independent variable (x).
DTPA concentrations (mg kg-1) varied widely in the ranges: 36–455 for Fe, 0.63–119 for Mn, 0.14–11.36 for Zn, 0.11–5.12 for Cu, 0.000–0.054 for Cr, 0.021–0.767 for Co, 0.169–1.413 for Ni, 0.008–0.180 for Cd, and 0.176–2.663 for Pb. Mehlich-3 concentrations (mg kg-1) varied widely in the ranges: 13–388 for Fe, 0.74–118 for Mn, 0.19–15.21 for Zn, 0.04–7.06 for Cu, 0.000–0.185 for Cr, 0.010–1.260 for Co, 0.106–0.949 for Ni, 0.000–0.186 for Cd, and 0.083–3.215 for Pb. As a general trend, the available metal concentrations decreased with depth, although the differences were not always significant (Figure 3). An exception is chromium extracted by Mehlich 3 reagent, which increased significantly with depth.
The fraction of the available metal in relation to the pseudo-total metal varied widely among the different metals, from negligible values for chromium to very high values for cadmium or lead (Supplementary Tables S4, S5). The ranges of variation were: 0.10%–1.07% for DTPA Fe, 0.04%–1.54% for Mehlich-3 Fe, 0.19%–12.65% for DTPA Mn, 0.19%–13.39% for Mehlich-3 Mn, 0.24%–39.60% for DTPA Zn, 0.40%–53.11% for Mehlich-3 Zn, 0.48%–49.19% for DTPA Cu, 0.21%–73.03% for Mehlich-3 Cu, 0.00%–0.13% for DTPA Cr, 0.00%–0.29% for Mehlich-3 Cr, 0.14%–18.48% for DTPA Co, 0.06%–30.36% for Mehlich-3 Co, 0.43%–18.19% for DTPA Ni, 0.34%–14.61% for Mehlich-3 Ni, 1.08%–61.45% for DTPA Cd, 0.00%–59.24% for Mehlich-3 Cd, 1.98%–43.49% for DTPA Pb, and 1.80%–58.07% for Mehlich-3 Pb. The values showed for all metals a trend to decrease with depth, that is, the fraction of available metal is highest at soil surface, except for Mehlich-3 Cr, which increased with depth. Significant differences among depths were found for the fractions of DTPA Fe, Mn, Zn, Ni and Cd and for the fractions of Mehlich-3 Fe, Mn, Zn, Cr, Ni and Cd.
The concentrations of metals soluble in 0.01M CaCl2 (Figure 3) were a tiny fraction of pseudo-total or available metals (Supplementary Table S6), ranging from 0 μg Pb kg−1, 0–2 µg Cd kg−1 or 0–4 µg Cr kg−1 to 0–339 µg Zn kg−1, 0–352 µg Fe kg−1 or 0–6,902 µg Mn kg−1. Iron and manganese were the most soluble metals, the soluble fraction being on average 0.16% and 0.17% of pseudo-total metal, respectively. The soluble fraction of cadmium was on average 0.35% of pseudo-total metal. Lead concentrations in the CaCl2 extracts were always below the limit of detection. The concentrations of metals soluble in 0.01M CaCl2 often correlated significantly with DTPA and/or Mehlich 3 extractions. Correlations with DTPA extractions are shown in Table 6. Only in the case of Cr significant (p < 0.05) differences in soluble metal were observed among soil depths (Figure 3).
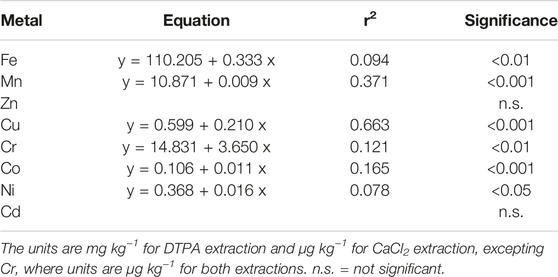
TABLE 6. Regression equations between CaCl2 and DTPA extractions. The concentration determined by DTPA extraction is the dependent variable (y) and that determined by CaCl2 extraction is the independent variable (x).
Discussion
Pseudo-Total Metal Concentrations and Pollution Indices
The pseudo-total iron concentrations were below or very close to the average iron concentration in the Earth’s crust, and the iron geoaccumulation indices (Igeo) were always negative. This indicates the absence of iron pollution. Thus, iron will be considered mainly in its micronutrient condition. The concentrations were higher than the total concentrations, determined by X-ray fluorescence, reported by Seco-Reigosa et al. (2015) for four pasture soils of the same area. In the case of manganese, 39% of the samples analysed, mainly in the surface soil, had a pseudo-total concentration higher than the GRL. The values were higher than those reported by Seco-Reigosa et al. (2015) for pasture and forest soils of the same area and similar to those reported by Caridad-Cancela et al. (2002) for natural soils in Galicia. Moreover, the maximum allowable concentration range for Mn (1,500–3,000 mg kg−1, according to Kabata-Pendias and Szteke (2015)) was never exceeded. The EF values of 14% of the samples indicated moderate enrichment and that of one sample (out of 80) indicated significant enrichment. The Igeo values indicated an unpolluted to moderately polluted situation in 18% of the samples. Although Mn is a relatively abundant element in the Earth’s crust, the data indicate in some cases a moderate anthropogenic enrichment, which can be related to the application of slurry or inorganic fertilisers. Slurry from the river Magdalena basin has a pseudo-total Mn concentration of 574 ± 138 mg kg−1 dry matter (Calvo-Rodríguez, 2003). Given that manganese is not a highly toxic element, the situation relative to this element in the river Magdalena basin does not appear to be of concern.
Thirty two percent of the samples analysed presented pseudo-total zinc concentrations higher than the edaphogeochemical background for Galician soils (100 mg kg−1), but the values were always much lower than the risk level (300 mg kg−1) and lower than the maximum allowable concentration (MAC) range for agricultural soils (100–300 mg kg−1) according to Kabata-Pendias (2011). Twelve percent of the samples analysed exceeded the edaphogeochemical background for copper in Galician soils (45 mg kg−1) and three samples (4%) exceeded the risk level (50 mg kg−1) but did not exceed the MAC range for agricultural soils (60–150 mg kg−1) according to Kabata-Pendias (2011). Most samples presented zinc and copper EFs lower than 2 and negative Igeo values. Three samples presented zinc EF slightly higher than 2, while two samples presented copper EF slightly higher than 2 (moderate enrichment). One sample presented a slightly positive copper Igeo (0.09). These results support the mixed lithogenic and anthropogenic nature of Zn and Cu in the soils of the river Magdalena basin (Franco-Uria et al., 2009). The strong correlation between the pseudo-total Zn and Cu concentrations (Supplementary Table S1) is in accordance with a common origin of these elements. In a similar way, Sterckeman et al. (2006) reported the association of Zn and Cu in soils developed from sedimentary materials in Northern France. The concentrations of zinc and copper are moderate in the cattle slurry from farms in the river Magdalena basin (260 ± 117 mg Zn kg−1 dry matter and 43 ± 18 mg Cu kg−1 dry matter (Calvo-Rodríguez, 2003)), although low compared to published values (300–410 mg Zn kg−1 and 74–100 mg Cu kg−1) for Dutch farms (Jakubus et al., 2013). These values are well below the maximum concentrations of Zn and Cu (2,500 mg Zn kg−1 and 1,000 mg Cu kg−1) in materials allowed to be applied to agricultural soils according to the Spanish legislation, even though manure is excluded from this limitation (MINISTERIO DE LA PRESIDENCIA, 2022). Despite the Zn and Cu contribution from slurry, the soils of the basin did not show significant enrichment or contamination by these metals. Unlike our results, Seco-Reigosa et al. (2015) reported values of the Zn and Cu total concentrations below the GRL. The pseudo-total concentrations of Zn and Cu in the present study were comparable to those reported by Paz-González et al. (2000), Caridad-Cancela et al. (2002) and Lopez-Mosquera et al. (2005) for Galician soils.
Twelve percent of the samples exceeded the GRL for Cr (85 mg kg−1). Seco-Reigosa et al. (2015) reported Cr concentrations above the GRL for pasture and forest soils of the same area. The GRL for Co and Ni (40 and 80 mg kg−1, respectively) were never exceeded, consistently with Seco et al. (Seco-Reigosa et al., 2015). The MAC ranges for Cr and Co in agricultural soils according to Kabata-Pendias (2011) (50–200 and 20–50 mg kg−1, respectively) were not exceeded either, while the MAC range for Ni in agricultural soils (20–60 mg kg−1) was exceeded by one sample (20–40 cm depth). In soils with a pH below 7, Cr is found predominantly in the form of Cr3+ (Bartlett and Kimble, 1976), a much less mobile and less toxic form than CrO42- (Fendorf, 1995). The EF values for these three elements indicated minimum enrichment in all cases, consistently with the situation reported by Sterckeman et al. (2006) for soils derived from sediments in France, while the Igeo values indicated a situation of no pollution. These results are in accordance with the identification of a lithogenic origin for these elements in the river Magdalena basin (Franco-Uria et al., 2009). The significant increases of Ni pseudo-total concentration and EF with depth (Figure 2; Table 3) also indicate a lithological origin. Similarly, the (not significantly) higher values at depth of Cr and Co concentrations, EF and Igeo and Ni Igeo are in accordance with the lithological origin of these metals. Moreover, this origin is supported by the strong bivariate correlations of the pseudo-total concentrations of Ni, Cr and Co with those of Fe, a lithogenic element (Supplementary Table S1). Consistent with our results, Cr, Co, and Ni were associated with Fe and Al in soils developed from sedimentary materials in northern France (Sterckeman et al., 2006). The pseudo-total concentrations of Cr, Co and Ni were comparable to those reported by Paz-González et al. (2000), Lopez-Mosquera et al. (2005) and Seco-Reigosa et al. (2015) for Galician soils.
Cadmium was the element whose pseudo-total concentrations most frequently exceeded the edaphogeochemical background for Galician soils (0.25 mg kg−1; 56% of the analysed samples), only one sample exceeded the risk level (2 mg kg−1), and the MAC range for agricultural soils (1–5 mg kg−1) according to Kabata-Pendias (2011) was never exceeded. The values were in accordance with those reported by Lopez-Mosquera et al. (2005) for pasture soils of Galicia. Sterckeman et al. (2006) reported Cd total concentrations in the range 0.01–8.98 mg kg−1 for soils derived from sediments in France. EF indicating moderate enrichment were found in 27% of samples, while 5% of samples (all depths from one soil) showed significant enrichment. The Igeo indicated unpolluted to moderately polluted soils in 27% of samples, moderately polluted soil in one sample and moderately to strongly polluted soil at all depths of the soil presenting significant enrichment. Cd is an element very toxic to plants, animals and humans (Alvarez-Ayuso, 2008) and has an anthropogenic origin in the river Magdalena basin (Franco-Uria et al., 2009). It is rather mobile and available in soils, having a high potential of transfer from soil to plants (McLaughlin et al., 1996; Pereira et al., 2011). The cadmium concentrations in the slurry from the basin were 23.4 ± 4.7 mg kg−1 dry matter (Calvo-Rodríguez, 2003), what is higher than the maximum concentration (10 mg Cd kg−1 dry matter) in materials allowed to be applied to agricultural soils according to the Spanish legislation (MINISTERIO DE LA PRESIDENCIA, 2022), although manure is excluded from this limitation. Furthermore, Cd is contributed to soil with inorganic phosphate fertilisers (Williams and David, 1976; McLaughlin et al., 1996; Nziguheba and Smolders, 2008; Cakmak et al., 2010). The pseudo-total Cd concentrations in the present study were slightly higher than those reported by Andrews et al. (1996) and Loganathan and Hedley (1997) for New Zealand pasture soils with a phosphate fertilisation history. According to McLaughlin et al. (1996) and Loganathan et al. (2008), Cd is the element of most concern among the contaminants present as impurities in inorganic fertilisers. As stated by Sterckeman et al. (2006), the high Cd concentrations can also be related to Cd enrichment of sedimentary parent materials relative to the average Earth’s crust. The significantly higher Cd Igeo in surface soil (Table 4), as well as the (non-significant) trend to decrease at depth of Cd concentration and EF (Figure 2; Table 3), are in accordance with the anthropogenic origin of this metal. The Cd enrichment in surface soils is consistent with the results for soils developed from sediments in northern France (Sterckeman et al., 2006), including forest, agriculture and grassland soils. That study reported higher enrichment in permanent grassland than in cultivation or forest soils. Contrary to Loganathan and Hedley (1997) and Loganathan et al. (2008), that reported limited Cd downward movement in pastoral soils, in the present study enrichment factors above 2 and positive geoaccumulation indices were found in a few soils at a depth of 20–40 cm. In contrast to Loganathan and Hedley (1997) and Loganathan et al. (2008) results, Sterckeman et al. (2000) found traces of Cd and Zn contamination at a depth of 2 m in the vicinity of lead and zinc smelters and reported that Cd is more mobile than Pb and Zn. The Cd mobility is attributed to the rather weak adsorption to silicate clays, oxides and organic matter in acid soils (Sterckeman et al., 2006; Alvarez-Ayuso, 2008; Pereira et al., 2011). Only one soil in the present study showed high pseudo-total Cd concentrations at all depths (1.64–2.12 mg kg−1, displayed as extreme values in Figure 2), close to the risk level, with enrichment factors between 6.72 and 8.70 (significant enrichment) and Igeo values between 2.13 and 2.50 (moderately to strongly polluted). Excepting this soil, the Cd pseudo-total concentrations were below 1 mg kg−1. There is no currently generalised risk associated to Cd pollution in the basin. However, if the application of slurry and superphosphate continues, the cadmium build-up may grow. Moreover, there is a risk of downward movement of Cd. It is necessary to monitor the concentration of cadmium in the soil and to determine cadmium in the fertilisers applied, limiting the application of slurry and avoiding the application of cadmium-rich phosphates.
The pseudo-total lead concentrations were always below the edaphogeochemical background for Galician soils (55 mg kg−1) and the MAC for agricultural soils (20–300 mg kg−1; Kabata-Pendias, 2011). The EF always indicated minimum enrichment, while the Igeo indicated that the soils were practically unpolluted. Although Pb in soils is mainly anthropogenic (Franco-Uria et al., 2009; Kabata-Pendias, 2011), the rural nature of the river Magdalena basin explains the absence of Pb pollution. The concentrations were similar to those reported by Lopez-Mosquera et al. (2005) and Seco-Reigosa et al. (2015) and lower than some of the values presented by Paz-González et al. (2000) for agricultural topsoils in Galicia, Spain.
The pseudo-total Zn, Cu and Pb concentrations at 0–5 cm depth were lower than those reported by Guo et al. (2018) for the same depth in Chinese crop soils treated with pig manure. Ni concentrations were slightly lower, while Cr and Cd concentrations were similar to those reported by Guo et al. (2018).
Available and Soluble Metal Concentrations
The total element concentrations in soils do not allow predicting their mobility and plant availability, which depend on soil properties such as pH, clay content and type, contents of Al, Fe and Mn oxyhydroxides or organic matter. In the short and medium term, metals extracted by appropriate reagents are a better estimation of their bioavailability. For trace metals, EDTA (Trierweiler and Lindsay, 1969), DTPA (Lindsay and Norvell, 1978) and Mehlich 3 (Mehlich, 1984) are reagents usually employed to extract bioavailable forms. CaCl2 solutions can be used to estimate soluble forms (Lebourg et al., 1996). These reagents are useful to assess the availability of micronutrients to vegetation and the risk associated to the presence of toxic elements in soils. Some studies reveal medium to good correlations between the concentrations of trace metals determined with DTPA and Mehlich 3 reagents. Their use in the present study aims to compare both extractions and check if there is a good correlation between the concentrations of trace metals determined with both reagents in acid soils of NW Spain.
The concentrations of DTPA Fe (Figure 3) were always well above the threshold value of 4.5 mg kg−1 reported by Lindsay and Norvell (1978) for Fe deficiency. Similarly, the concentrations of DTPA Mn were well above the threshold value of 0.2 mg kg−1 reported by Sims and Johnson (1991). The Zn concentrations at 0–10 cm depth were above the threshold value of 0.8 mg kg−1 (Lindsay and Norvell, 1978), but were often below this value at 10–20 and especially at 20–40 cm depth. The Cu concentrations at 0–5 cm depth were above the threshold value of 0.2 mg kg−1 (Lindsay and Norvell, 1978); at 5–10, 10–20 and 20–40 cm depth, the Cu concentrations were below the threshold value in two to four soils.
The Mehlich-3 Mn concentrations (Figure 3) were always above the deficiency threshold of 3.9 mg kg−1 (Sims and Johnson, 1991) in 0–5 and 5–10 cm samples and in most 10–20 and 20–40 cm samples. The Mehlich-3 Zn concentrations were always above the critical deficiency range of 1.15–1.62 mg kg−1 (Sims and Johnson, 1991) in 0–5 and 5–10 cm samples and often in 10–20 cm samples, while the samples at 20–40 cm depth were often below this threshold. For Mehlich-3 Cu, Sims and Johnson (1991) reported the critical deficiency range of 1–10 mg kg−1. The value of 1 mg kg−1 was exceeded by most of the 0–5 cm samples, while at higher depths most samples were below this value.
In short, the micronutrients Fe and Mn were available at sufficiency levels in most of the studied soils at all depths (0–40 cm), while Zn and Cu were at sufficiency levels at 0–5 cm and below these levels with increasing frequency at higher depths.
The critical level of 200 mg kg−1 extracted by DTPA reported by Monterroso et al. (1999) for Fe toxicity was exceeded by six samples at 0–5 cm depth and with decreasing frequency at 5–10 and 10–20 cm depth (Figure 3). The critical level of 60 mg kg−1 for Mn toxicity (Monterroso et al., 1999) was exceeded by six samples at 0–5 cm depth and by two samples at 5–10 cm depth.
The critical level of toxicity for Zn (2 mg kg−1, Sims and Johnson, 1991) was exceeded by all the soils analysed at a depth of 0–5 cm and with decreasing frequency at greater depths. For Cu, the toxicity threshold of 2 mg kg−1 (Sims and Johnson, 1991) was exceeded by two soils at all depths (Figure 3).
The available Ni and Pb concentrations were within the ranges of critical levels of toxicity (0.03–5.34 mg kg−1 for DTPA Ni; 0.04–5.93 mg kg−1 for Mehlich-3 Ni; 0.44–39 mg kg−1 for DTPA Pb; 0.66–29 mg kg−1 for Mehlich-3 Pb) reported by Silva et al. (2020), despite the lithogenic origin of these elements and the absence of pollution indicated by the total concentrations and the pollution indices. However, according to Silva et al. (2020), the critical levels for these elements vary widely among soils and might not apply to the soils in the present study. Therefore, the absence of Ni and Pb pollution appears most plausible.
The available Cd concentrations (extracted either by DTPA or Mehlich 3) were above or close to the critical levels of toxicity (0.01–0.04 mg kg−1) reported by Silva et al. (2020). However, contrary to pseudo-total Cd (Figure 2), the concentrations of DTPA and Mehlich-3 Cd (Figure 3) decreased significantly with depth, indicating a decreased risk by Cd pollution.
In summary, the toxicity thresholds for Fe and Mn were exceeded by a few samples, mainly at low depths. Given that these metals are not highly toxic, the situation regarding these elements does not seem worrisome, as already indicated by the data on pseudo-total concentrations and the values of the pollution indices. The toxicity threshold for Zn was often exceeded, mainly at low depths. It is worth highlighting the short distance between published Zn deficiency and toxicity thresholds. Taking into account the rapid decrease with depth (Figure 3), the situation does not seem worrisome for Zn either. As for Cu, only two soils could be at risk of toxicity (Figure 3). No risk of Ni or Pb toxicity appears either. As for Cd, the results show decreased toxicity with depth.
The significant decrease with depth in the concentrations of most metals in available form (Figure 3; Supplementary Tables S4, S5) points to a greater availability of metals of anthropogenic origin (abundant in surface soils), while metals of lithological origin (abundant in deep soils) would be largely in non-available form. An exception is Mehlich-3 Cr, which increases with depth, in a way similar to pseudo-total Cr (Figure 2), although it is a very small fraction of the pseudo-total Cr. In fact, the Cr presented a very low availability in all samples. The opposite variations with depth of DTPA Cr and Mehlich-3 Cr indicate that these reagents extract different pools of Cr.
The Mehlich 3 reagent extracted more Fe, Zn, Cu, Co and Pb, less Ni and similar quantities of Mn and Cd compared to DTPA. According to Monterroso et al. (1999), the acidity of Mehlich 3 reagent (pH 3) is responsible for its higher extracting power. The regression equations in Table 5 allow to estimate the DTPA concentrations from the Mehlich-3 concentrations (or vice-versa), the Mehlich 3 extraction being less time-consuming and allowing the determination of available macronutrients in the same extract. The lack of significant correlation between DTPA Cr and Mehlich-3 Cr is in accordance with the fact that the two reagents extract different pools of chromium.
Significant bivariate correlations, in most cases positive, were found among different metals extracted either by DTPA or Mehlich 3 reagent (Supplementary Tables S2, S3), suggesting associations between the metals presenting the strongest correlations. It is worth mentioning that DTPA Cr correlated significant and positively with most other metals (excepting Fe and Pb), while Mehlich-3 Cr correlated negatively with most other metals. This is consistent with the fact that these reagents extract different pools of Cr and suggests that DTPA is more suitable than Mehlich 3 to estimate available Cr.
The available metal concentrations (determined either by DTPA or Mehlich 3) as percent of pseudo-total concentrations (Supplementary Tables S4, S5) indicate that most chromium is in a non-available form, while a considerable proportion of Cd and Pb are in available form.
The available Zn, Cu, Co, Ni and Pb were comparable to the values reported by Paz-González et al. (2000) for the same metals extracted by EDTA from agricultural topsoils in Galicia, Spain.
The DTPA and Mehlich-3 extracted Fe, Mn, Zn and Cu were similar or slightly higher than those reported by Caridad-Cancela et al. (2002) for the same elements extracted by the same reagents from natural soils of Galicia, Spain. This is consistent with the fact that the soils of the river Magdalena basin are representative of Galician pasture soils.
The DTPA Zn, Cu and Pb concentrations at 0–5 cm depth were much lower, those of Cd and Ni were lower and those of Cr were similar to those reported by Guo et al. (2018) for the same depth in Chinese crop soils treated with pig manure, in accordance with the much lower Cu and Zn concentrations in cattle manures compared with pig manures.
The analysis of soluble metals (extractable with 1 M CaCl2) will provide complementary information on the downward movement of heavy metals. As expected, 0.01 M CaCl2 extracted much less metals than DTPA and Mehlich 3 reagents (Figure 3). Fe, Mn and Cd were the elements extracted in highest proportion (Supplementary Table S6). The variation with depth is significant only in the case of chromium.
CaCl2 has been suggested as the best predictor of phytoavailable metals, especially Cd, in soils (Whitten and Ritchie, 1991; Andrews et al., 1996; Lebourg et al., 1996; McLaughlin et al., 2000; Adamo et al., 2018). The CaCl2-extractable Cd concentrations were well below the critical value of 80 μg kg−1 proposed by Podlešáková et al. (2002). Despite the slightly higher concentrations of pseudo-total Cd, the soils in the present study had much lower concentrations of Cd extracted by 0.01 M CaCl2 than those reported by Andrews et al. (1996) and Loganathan and Hedley (1997) for New Zealand pasture soils with a phosphate fertilisation history and similar pH and organic carbon (0.009–0.034 mg Cd kg-1). This suggests that Cd in the soils of the river Magdalena basin is much less available than in the New Zealand soils studied by Andrews et al. (1996) and Loganathan and Hedley (1997). It is worth noticing that in those New Zealand soils the reported values of Cd extracted by EDTA are very close to total concentrations. Cadmium contributed by cattle slurry seems to be less mobile than that supplied by soluble phosphate fertilisers. Contrary to other forms of Cd, the concentrations of Cd extracted by 0.01 M CaCl2 indicate that there is no risk of cadmium transfer from soil to plants or to water bodies. Chelating agents, such as DTPA or Mehlich-3 reagent, can extract organically bound metals and metals specifically adsorbed by the clay fraction, so overestimating the bioavailable fraction of some elements (Adamo et al., 2018).
In a similar way to Cd, the CaCl2-extracted Mn, Zn, Cu, Co, Cr, Ni and Pb were well below the critical values proposed by Podlešáková et al. (2002) (30, 2, 0.2, 0.5, 0.05, 100 and 7.5 mg kg−1, respectively). This confirms the general non-problematic situation regarding these metals indicated by the results of total and available concentrations.
The values of pseudo-total Cd as well as the cadmium extracted by chelating agents make it advisable to limit the contribution of phosphate fertilisers in coarser textured soils and to apply limestone as an amendment to reduce the cadmium mobility in those soils with higher concentrations (Andrews et al., 1996; Loganathan and Hedley, 1997; Gray et al., 1999; Alvarez-Ayuso, 2008).
Conclusion
The study reveals the absence of pollution by the lithogenic Fe, Ni, Co and Cr. Most samples presented minimum enrichment in the mixed-origin Mn, Zn and Cu, while a few samples showed moderate enrichment, resulting in unpolluted to moderately polluted soils. The soils of the river Magdalena basin were unpolluted by Pb.
Cadmium was the element that most frequently presented situations of pollution (moderate in most cases), revealed both by the values of pseudo-total and available (extracted by DTPA or Mehlich 3) concentrations. Furthermore, it appeared to be moderately mobile in these acid soils, even though the concentrations in available form decreased more significantly with depth than the pseudo-total concentrations. Despite the high values of pseudo-total Cd and Cd extracted by chelating agents, the extraction by 0.01 M CaCl2 pointed to very low environmental risk related to Cd pollution.
The micronutrients Fe and Mn were at sufficient levels for plant growth, while Zn and Cu were above the sufficiency level in the surface soil (0–5 or 0–10 cm) but deficiency situations might appear in some cases below 10 cm depth.
It is shown that the application of cattle slurry to grassland soils in Galicia, NW Spain, does not entail an environmental risk associated to the contribution of toxic metals, excepting Cd, for which precautions are advised to avoid possible future risk situations in the event that cadmium continues to be added to the soil.
Data Availability Statement
The raw data supporting the conclusion of this article will be made available by the authors, without undue reservation.
Author Contributions
Conceptualization: MF-M and FD-R. Methodology: MF-M and FD-R. Soil sampling: CL-M, MF-M, and FD-R. Samples processing and laboratory analysis: CL-M and RM-R. Data analysis: CL-M, MF-M, and FD-R. Writing: MF-M. Funding acquisition: MF-M and FD-R.
Funding
This work has been supported by the Spanish Ministry of Education (Project CTM2004-07258/TECNO) and the Dirección Xeral de I+D (Xunta de Galicia) (Project FARIA, PGDIT05TAM00201CT).
Conflict of Interest
The authors declare that the research was conducted in the absence of any commercial or financial relationships that could be construed as a potential conflict of interest.
Acknowledgments
The authors wish to thank two reviewers for their helpful comments.
Supplementary Material
The Supplementary Material for this article can be found online at: https://www.frontierspartnerships.org/articles/10.3389/sjss.2023.11201/full#supplementary-material
Supplementary Data Sheet 1 | Supplementary Tables.
Supplementary Data Sheet 2 | Analytical methods.
References
Acevedo-Figueroa, D., Jimenez, B. D., and Rodriguez-Sierra, C. J. (2006). Trace Metals in Sediments of Two Estuarine Lagoons from Puerto Rico. Environ. Pollut. 141, 336–342. doi:10.1016/j.envpol.2005.08.037
Adamo, P., Agrelli, D., and Zampella, M. (2018). Chemical Speciation to Assess Bioavailability, Bioaccessibility and Geochemical Forms of Potentially Toxic Metals (PTMs) in Polluted Soils. Environ. Geochem. (Second Ed.) 2018, 153. doi:10.1016/B978-0-444-63763-5.00010-0
Aldrich, A. P., Kistler, D., and Sigg, L. (2002). Speciation of Cu and Zn in Drainage Water from Agricultural Soils. Environ. Sci. Technol. 36, 4824–4830. doi:10.1021/es025813x
Alloway, B. J., and Jackson, A. P. (1991). The Behaviour of Heavy Metals in Sewage Sludge-Amended Soils. Sci. Total Environ. 100, 151–176. doi:10.1016/0048-9697(91)90377-q
Alvarez-Ayuso, E. (2008). Cadmium in Soil-Plant Systems: an Overview. Int. J. Environ. Pollut. 33, 275–291. doi:10.1504/ijep.2008.019399
Andrews, P., Town, R. M., Hedley, M. J., and Loganathan, P. (1996). Measurement of Plant-Available Cadmium in New Zealand Soils. Aust. J. Soil Res. 34, 441–452. doi:10.1071/sr9960441
Antoniadis, V., Robinson, J. S., and Alloway, B. J. (2008). Effects of Short-Term pH Fluctuations on Cadmium, Nickel, Lead, and Zinc Availability to Ryegrass in a Sewage Sludge-Amended Field. Chemosphere 71, 759–764. doi:10.1016/j.chemosphere.2007.10.015
Antunes, S. C., Pereira, R., Marques, S. M., Castro, B. B., and Goncalves, F. (2011). Impaired Microbial Activity Caused by Metal Pollution: A Field Study in a Deactivated Uranium Mining Area. Sci. Total Environ. 410, 87–95. doi:10.1016/j.scitotenv.2011.09.003
Baize, D. (1997). Detection of Moderate Contamination by Trace Metals in Agricultural Soils. Analusis 25, M29–M35.
Bartlett, R. J., and Kimble, J. M. (1976). Behavior of Chromium in Soils: I. Trivalent Forms. J. Environ. Qual. 5, 379–383. doi:10.2134/jeq1976.00472425000500040009x
Batley, G. E. (2012). Heavy Metal"-Aa Useful Term. Integr. Environ. Assess. Manag. 8, 215. doi:10.1002/ieam.1290
Buat-Menard, P., and Chesselet, R. (1979). Variable Influence of the Atmospheric Flux on the Trace-Metal Chemistry of Oceanic Suspended Matter. Earth Planet. Sci. Lett. 42, 399–411. doi:10.1016/0012-821x(79)90049-9
Cakmak, D., Saljnikov, E., Mrvic, V., Jakovljevic, M., Marjanovic, Z., Sikiric, B., et al. (2010). Soil Properties and Trace Elements Contents Following 40 Years of Phosphate Fertilization. J. Environ. Qual. 39, 541–547. doi:10.2134/jeq2009.0216
Calvo-Rodríguez, F. (2003). Caracterización dos puríns de vacuno das explotacións da conca do río Magdalena (A Pastoriza). Master Thesis Dissertation (Santiago, Spain: University of Santiago de Compostela).
Caridad-Cancela, R., de Abreu, C. A., and Paz-Gonzalez, A. (2002). DTPA and Mehlich-3 Micronutrient Extractability in Natural Soils. Commun. Soil Sci. Plant Analysis 33, 2879–2893. doi:10.1081/css-120014488
Daskalakis, K. D., and Oconnor, T. P. (1995). Normalization and Elemental Sediment Contamination in the Coastal United States. Environ. Sci. Technol. 29, 470–477. doi:10.1021/es00002a024
de la Torre, A. I., Jimenez, J. A., Carballo, M., Fernandez, C., Roset, J., and Munoz, M. J. (2000). Ecotoxicological Evaluation of Pig Slurry. Chemosphere 41, 1629–1635. doi:10.1016/s0045-6535(00)00038-2
de Temmerman, L., Vanongeval, L., Boon, W., Hoenig, M., and Geypens, M. (2003). Heavymetal Content of Arable Soils in Northern Belgium. Water Air Soil Pollut. 148, 61–76. doi:10.1023/a:1025498629671
Del Castilho, P., Chardon, W. J., and Salomons, W. (1993). Influence of Cattle-Manure Slurry Application on the Solubility of Cadmium, Copper, and Zinc in a Manured Acidic, Loamy-Sand Soil. J. Environ. Qual. 22, 689–697. doi:10.2134/jeq1993.00472425002200040009x
Duffus, J. H. (2002). "Heavy Metals" - A Meaningless Term? (IUPAC Technical Report). Pure Appl. Chem. 74, 793–807. doi:10.1351/pac200274050793
Fendorf, S. E. (1995). Surface-reactions of Chromium in Soils and Waters. Geoderma 67, 55–71. doi:10.1016/0016-7061(94)00062-f
Fernandez-Calvino, D., Soler-Rovira, P., Polo, A., Diaz-Ravina, M., Arias-Estevez, M., and Plaza, C. (2010). Enzyme Activities in Vineyard Soils Long-Term Treated with Copper-Based Fungicides. Soil Biol. Biochem. 42, 2119–2127. doi:10.1016/j.soilbio.2010.08.007
Franco-Uria, A., Lopez-Mateo, C., Roca, E., and Fernandez-Marcos, M. L. (2009). Source Identification of Heavy Metals in Pastureland by Multivariate Analysis in NW Spain. J. Hazard. Mater. 165, 1008–1015. doi:10.1016/j.jhazmat.2008.10.118
Gray, C. W., Mclaren, R. G., Roberts, A. H. C., and Condron, L. M. (1999). Effect of Soil pH on Cadmium Phytoavailability in Some New Zealand Soils. N. Z. J. Crop Hortic. Sci. 27, 169–179. doi:10.1080/01140671.1999.9514093
Guo, T., Lou, C. L., Zhai, W. W., Tang, X. J., Hashmi, M. Z., Murtaza, R., et al. (2018). Increased Occurrence of Heavy Metals, Antibiotics and Resistance Genes in Surface Soil after Long-Term Application of Manure. Sci. Total Environ. 635, 995–1003. doi:10.1016/j.scitotenv.2018.04.194
Gupta, S. K., Vollmer, M. K., and Krebs, R. (1996). The Importance of Mobile, Mobilisable and Pseudo Total Heavy Metal Fractions in Soil for Three-Level Risk Assessment and Risk Management. Sci. Total Environ. 178, 11–20. doi:10.1016/0048-9697(95)04792-1
Gustin, M. S., Hou, D., and Tack, F. M. G. (2021). The Term "heavy Metal(s)": History, Current Debate, and Future Use. Sci. Total Environ. 789, 147951. doi:10.1016/j.scitotenv.2021.147951
Hodson, M. E. (2004). Heavy Metals - Geochemical Bogey Men? Environ. Pollut. 129, 341–343. doi:10.1016/j.envpol.2003.11.003
Houba, V. J. G., Novozamsky, I., Lexmond, T. M., and Vanderlee, J. J. (1990). Applicability of 0.01 M CaCl2 as a Single Extraction Solution for the Assessment of the Nutrient Status of Soils and Other Diagnostic Purposes. Commun. Soil Sci. Plant Analysis 21, 2281–2290. doi:10.1080/00103629009368380
IUPAC (2022). Trace element [Online]. Available at: https://goldbook.iupac.org/terms/view/T06421 (Accessed Sepember, 2022).
IUSS Working Group WRB (2022). World Reference Base for Soil Resources. International Soil Classification System for Naming Soils and Creating Legends for Soil Maps. 4th ed. Vienna, Austria: International Union of Soil Sciences (IUSS).
Jakubus, M., Dach, J., and Starmans, D. (2013). Bioavailability of Copper and Zinc in Pig and Cattle Slurries. Fresenius Environ. Bull. 22, 995–1002.
Kabata-Pendias, A., and Szteke, B. (2015). Trace Elements in Abiotic and Biotic Environments. Abingdon-on-Thames, Oxfordshire, UK: Taylor & Francis.
L'Herroux, L., Leroux, S., Appriou, P., and Martinez, J. (1997). Behaviour of Metals Following Intensive Pig Slurry Applications to a Natural Field Treatment Process in Brittany (France). Environ. Pollut. 97, 119–130. doi:10.1016/s0269-7491(97)00072-9
Lebourg, A., Sterckeman, T., Ciesielski, H., and Proix, N. (1996). Intérêt de différents réactifs d'extraction chimique pour l'évaluation de la biodisponibilité des métaux en traces du sol. Agronomie 16, 201–215. doi:10.1051/agro:19960401
Li, Y., Xu, Z. Q., Ren, H. H., Wang, D., Wang, J., Wu, Z., et al. (2022). Spatial Distribution and Source Apportionment of Heavy Metals in the Topsoil of Weifang City, East China. Front. Environ. Sci. 10. doi:10.3389/fenvs.2022.893938
Liang, C. N., and Tabatabai, M. A. (1977). Effects of Trace Elements on Nitrogen Mineralisation in Soils. Environ. Pollut. 12, 141–147. doi:10.1016/0013-9327(77)90017-9
Lindsay, W. L., and Norvell, W. A. (1978). Development of a DTPA Soil Test for Zinc, Iron, Manganese, and Copper. Soil Sci. Soc. Am. J. 42, 421–428. doi:10.2136/sssaj1978.03615995004200030009x
Loganathan, P., and Hedley, M. J. (1997). Downward Movement of Cadmium and Phosphorus from Phosphatic Fertilisers in a Pasture Soil in New Zealand. Environ. Pollut. 95, 319–324. doi:10.1016/s0269-7491(96)00142-x
Loganathan, P., Hedley, M. J., and Grace, N. D. (2008). Pasture Soils Contaminated with Fertilizer-Derived Cadmium and Fluorine: Livestock Effects. Rev. Environ. Contam. Toxicol. 192, 29. doi:10.1007/978-0-387-71724-1_2
Lopez-Mosquera, M. E., Barros, R., Sainz, M. J., Carral, E., and Seoane, S. (2005). Metal Concentrations in Agricultural and Forestry Soils in Northwest Spain: Implications for Disposal of Organic Wastes on Acid Soils. Soil Use Manag. 21, 298–305. doi:10.1079/sum2005324
Macías-Vázquez, F., and Calvo de Anta, R. (2009). Niveles genéricos de referencia de metales pesados y otros elementos traza en suelos de Galicia. Santiago de Compostela, Xunta de Galicia: Consellería de Medio Ambiente e Desenvolvemento Sostible.,
Madrid, L. (2010). Heavy Metals": Reminding a Long-Standing and Sometimes Forgotten Controversy. Geoderma 155, 128–129. doi:10.1016/j.geoderma.2009.11.031
Mantovi, P., Bonazzi, G., Maestri, E., and Marmiroli, N. (2003). Accumulation of Copper and Zinc from Liquid Manure in Agricultural Soils and Crop Plants. Plant Soil 250, 249–257. doi:10.1023/a:1022848131043
Mcbride, M. B. (1989). “Reactions Controlling Heavy Metal Solubility in Soils,” in Advances in Soil Science. Editor B. A. Stewart (New York, NY: Springer New York), Vol. 10.
Mclaughlin, M. J., Tiller, K. G., Naidu, R., and Stevens, D. P. (1996). Review: The Behaviour and Environmental Impact of Contaminants in Fertilizers. Aust. J. Soil Res. 34, 1–54. doi:10.1071/sr9960001
Mclaughlin, M. J., Zarcinas, B. A., Stevens, D. P., and Cook, N. (2000). Soil Testing for Heavy Metals. Commun. Soil Sci. Plant Analysis 31, 1661–1700. doi:10.1080/00103620009370531
Mehlich, A. (1984). Mehlich 3 Soil Test Extractant: A Modification of Mehlich 2 Extractant. Commun. Soil Sci. Plant Analysis 15, 1409–1416. doi:10.1080/00103628409367568
Meteogalicia, C. D. M. A. (2022). Xunta de Galicia, Spain. Available at: http://www.meteogalicia.gal (Accessed September, 2022).
Ministerio de la Presidencia, R. C. L. C. Y. M. D. (2022). Real Decreto 1051/2022, de 27 de diciembre, por el que se establecen normas para la nutrición sostenible en los suelos agrarios. Madrid, Spain: Boletín Oficial Del Estado.
Monterroso, C., Alvarez, E., and Marcos, M. L. F. (1999). Evaluation of Mehlich 3 Reagent as a Multielement Extractant in Mine Soils. Land Degrad. Dev. 10, 35–47. doi:10.1002/(sici)1099-145x(199901/02)10:1<35::aid-ldr319>3.0.co;2-6
Moreno-Caselles, J., Moral, R., Perez-Murcia, M., Perez-Espinosa, A., and Rufete, B. (2002). Nutrient Value of Animal Manures in Front of Environmental Hazards. Commun. Soil Sci. Plant Analysis 33, 3023–3032. doi:10.1081/css-120014499
Müller, G. (1979). Heavy Metals in the Sediments of the Rhine - Changes since 1971. Umschau Wissenschaft Und Tech. 79, 778–783.
Nagajyoti, P. C., Lee, K. D., and Sreekanth, T. V. M. (2010). Heavy Metals, Occurrence and Toxicity for Plants: a Review. Environ. Chem. Lett. 8, 199–216. doi:10.1007/s10311-010-0297-8
Nicholson, F. A., Smith, S. R., Alloway, B. J., Carlton-Smith, C., and Chambers, B. J. (2003). An Inventory of Heavy Metals Inputs to Agricultural Soils in England and Wales. Sci. Total Environ. 311, 205–219. doi:10.1016/s0048-9697(03)00139-6
Nieboer, E., and Richardson, D. H. S. (1980). The Replacement of the Non-descript Term Heavy-Metals by a Biologically and Chemically Significant Classification of Metal-Ions. Environ. Pollut. Ser. B-Chemical Phys. 1, 3–26. doi:10.1016/0143-148x(80)90017-8
Nunez-Delgado, A., Lopez-Periago, E., and Diaz-Fierros-Viqueria, F. (2002). Pollution Attenuation by Soils Receiving Cattle Slurry after Passage of a Slurry-like Feed Solution. Column Experiments. Bioresour. Technol. 84, 229–236. doi:10.1016/s0960-8524(02)00050-0
Nziguheba, G., and Smolders, E. (2008). Inputs of Trace Elements in Agricultural Soils via Phosphate Fertilizers in European Countries. Sci. Total Environ. 390, 53–57. doi:10.1016/j.scitotenv.2007.09.031
Paz-González, A., Taboada-Castro, T., and Taboada-Castro, M. (2000). Levels of Heavy Metals (Co, Cu, Cr, Ni, Pb, and Zn) in Agricultural Soils of Northwest Spain. Commun. Soil Sci. Plant Analysis 31, 1773–1783. doi:10.1080/00103620009370536
Pereira, B. F. F., Rozane, D. E., Araujo, S. R., Barth, G., Queiroz, R. J. B., Nogueira, T. A. R., et al. (2011). Cadmium Availability and Accumulation by Lettuce and Rice. Rev. Bras. De. Cienc. Do Solo 35, 645–654. doi:10.1590/s0100-06832011000200033
Podlešáková, E., Němeček, J., and Vácha, R. (2002). Critical Values of Trace Elements in Soils from the Viewpoint of the Transfer Pathway Soil - Plant. Plant, Soil Environ. 48, 193–202. doi:10.17221/4224-pse
Poulsen, P. H. B., Magid, J., Luxhoi, J., and de Neergaard, A. (2013). Effects of Fertilization with Urban and Agricultural Organic Wastes in a Field Trial - Waste Imprint on Soil Microbial Activity. Soil Biol. Biochem. 57, 794–802. doi:10.1016/j.soilbio.2012.02.031
Pourret, O., and Hursthouse, A. (2019). It's Time to Replace the Term "Heavy Metals" with "Potentially Toxic Elements" when Reporting Environmental Research. Int. J. Environ. Res. Public Health 16, 4446. doi:10.3390/ijerph16224446
Proshad, R., Islam, M. S., Kormoker, T., Sayeed, A., Khadka, S., and Idris, A. M. (2021). Potential Toxic Metals (PTMs) Contamination in Agricultural Soils and Foodstuffs with Associated Source Identification and Model Uncertainty. Sci. Total Environ. 789, 147962. doi:10.1016/j.scitotenv.2021.147962
Richter, R., Rimovsky, K., and Hlusek, J. (1997). The Productivity of a Crop Rotation under Conventional and Organic Method of Management in Conditions of Increased Content of Heavy Metals in the Soil. Acta Univ. Agric. Silvic. Mendelianae Brunensis 45, 83–90.
Rodriguez-Seijo, A., Andrade, M. L., and Vega, F. A. (2017). Origin and Spatial Distribution of Metals in Urban Soils. J. Soils Sediments 17, 1514–1526. doi:10.1007/s11368-015-1304-2
Romkens, P., and Salomons, W. (1998). Cd, Cu and Zn Solubility in Arable and Forest Soils: Consequences of Land Use Changes for Metal Mobility and Risk Assessment. Soil Sci. 163, 859–871. doi:10.1097/00010694-199811000-00003
Seco-Reigosa, N., Fernandez-Sanjurjo, M. J., Nunez-Delgado, A., Cutillas-Barreiro, L., Gomez-Armesto, A., Novoa-Munoz, J. C., et al. (2015). Heavy Metals in Pastureland Soils Situated in A Pastoriza (NW Spain) Treated with Cattle Slurry and NPK Fertilizers. Span. J. Soil Sci. 5, 154–164. doi:10.3232/sjss.2015.v5.n2.05
Silva, E. B., Alves, I. S., Alleoni, L. R. F., Grazziotti, P. H., Farnezi, M. M. M., Santos, L. L., et al. (2020). Availability and Toxic Level of Cadmium, Lead and Nickel in Contaminated Soils. Commun. Soil Sci. Plant Analysis 51, 1341–1356. doi:10.1080/00103624.2020.1763396
Sims, J. T., and Johnson, G. V. (1991). Micronutrient Soil Tests. Micronutr. Agric. 4. doi:10.2136/sssabookser4.2ed.c12
Smith, S. R. (1997). Rhizobium in Soils Contaminated with Copper and Zinc Following the Long-Term Application of Sewage Sludge and Other Organic Wastes. Soil Biol. Biochem. 29, 1475–1489. doi:10.1016/s0038-0717(97)00036-9
Sterckeman, T., Douay, F., Baize, D., Fourrier, H., Proix, N., and Schvartz, C. (2006). Trace Elements in Soils Developed in Sedimentary Materials from Northern France. Geoderma 136, 912–929. doi:10.1016/j.geoderma.2006.06.010
Sterckeman, T., Douay, F., Proix, N., and Fourrier, H. (2000). Vertical Distribution of Cd, Pb and Zn in Soils Near Smelters in the North of France. Environ. Pollut. 107, 377–389. doi:10.1016/s0269-7491(99)00165-7
Trierweiler, J. F., and Lindsay, W. L. (1969). EDTA-ammonium Carbonate Soil Test for Zinc. Soil Sci. Soc. Am. Proc. 33, 49–54. doi:10.2136/sssaj1969.03615995003300010017x
USEPA (2007). Method 3051A. Microwave Assisted Acid Digestion of Sediments, Sludges, Soils, and Oils [Online]. Washington, DC, USA: USEPA. Available at: https://www.epa.gov/hw-sw846/sw-846-test-method-3051a-microwave-assisted-acid-digestion-sediments-sludges-soils-and-oils (Accessed September, 2022).
Whitten, M. G., and Ritchie, G. S. P. (1991). Calcium-chloride Extractable Cadmium as an Estimate of Cadmium Uptake by Subterranean Clover. Aust. J. Soil Res. 29, 215–221. doi:10.1071/sr9910215
Wilcke, W., Bol, R., and Amelung, W. (2002). Fate of Dung-Applied Copper in a British Grassland Soil. Geoderma 106, 273–288. doi:10.1016/s0016-7061(01)00128-8
Williams, C. H., and David, D. J. (1976). Accumulation in Soil of Cadmium Residues from Phosphate Fertilizers and Their Effect on Cadmium Content of Plants. Soil Sci. 121, 86–93. doi:10.1097/00010694-197602000-00004
Xia, L., Lam, S. K., Yan, X., and Chen, D. (2017). How Does Recycling of Livestock Manure in Agroecosystems Affect Crop Productivity, Reactive Nitrogen Losses, and Soil Carbon Balance? Environ. Sci. Technol. 51, 7450–7457. doi:10.1021/acs.est.6b06470
Keywords: heavy metals, grassland soils, Mehlich-3 extraction, CaCl2 extraction, DTPA extraction, pastureland, microwave digestion, availability
Citation: López-Mateo C, Marcos-Rodríguez R, Díaz-Rodríguez F and Fernandez-Marcos ML (2023) Forms of Toxic and Trace Metals in Grassland Soils of Galicia, Spain. Span. J. Soil Sci. 13:11201. doi: 10.3389/sjss.2023.11201
Received: 16 January 2023; Accepted: 10 March 2023;
Published: 23 March 2023.
Edited by:
Avelino Núñez-Delgado, University of Santiago de Compostela, SpainCopyright © 2023 López-Mateo, Marcos-Rodríguez, Díaz-Rodríguez and Fernandez-Marcos. This is an open-access article distributed under the terms of the Creative Commons Attribution License (CC BY). The use, distribution or reproduction in other forums is permitted, provided the original author(s) and the copyright owner(s) are credited and that the original publication in this journal is cited, in accordance with accepted academic practice. No use, distribution or reproduction is permitted which does not comply with these terms.
*Correspondence: Maria Luisa Fernandez-Marcos, bWx1aXNhLmZlcm5hbmRlekB1c2MuZXM=