- 1Área de Edafoloxía e Química Agrícola, Facultade de Ciencias, Universidade de Vigo, Ourense, Spain
- 2Departamento de Bioquímica de Suelos, Ecosistemas y Ecología Agroforestal, Misión Biológica de Galicia (MBG-CSIC Sede Santiago), Santiago de Compostela, Spain
A laboratory experiment was carried out to investigate the response of the microbial communities in acid agricultural soils located in the NW Iberian Peninsula to the presence of clarithromycin. Four soils, with different organic C content and similar pH, and seven different concentrations of clarithromycin (0.49, 1.95, 7.81, 31.25, 125, 500 and 2,000 mg kg−1 of soil) were used, and microbial estimates were made after 8 and 42 incubation days. The phospholipid fatty acids (PLFA) technique was used to estimate the total microbial biomass and biomass of specific microbial groups as well as the microbial community structure (PLFA pattern). The microbial biomass (total and specific groups) was different in the four studied soils, the lowest values being exhibited by soils with the lowest organic C. The antibiotic addition showed a positive effect on microbial biomass (total and specific groups), especially at the highest dose; the effect being similar or even more accentuated with time passed after the addition (42 days ≥8 days). Principal component analysis (PCA) of the PLFA data carried out with the whole data set showed that the main determining factors of the microbial structure followed the order: soil > time incubation ≥ antibiotic dose. When the PCA was performed individually for each incubation time, the results indicated that microbial communities of the four soils were different. Likewise, for each soil, different microbial communities were observed depending on antibiotic concentration. The microbial biomass and PLFA pattern data were coincidentally showing that the clarithromycin addition favored fungi and G− bacteria more that bacteria and G+ bacteria; the effect being dose-dependent. Our data (microbial biomass, PLFA pattern) also demonstrated that the effect of clarithromycin addition on microbial communities in these four acid agricultural soils persisted even after 42 incubation days.
Introduction
Antibiotics, used worldwide for human health, are excreted in feces and urine as the origin compound and/or as secondary metabolites, reaching the environment and causing serious damage to the soil and aquatic ecosystems (Thiele-Bruhn, 2003; Kümmerer, 2009). These emergent contaminants of human origin, which are present in wastewater destined for wastewater treatment plants (WWTPs), can enter into soil following both agricultural irrigation with liquid effluents and the application of solid effluents (sewage sludge) as organic amendments to the soil (Fijalkowski et al., 2017; Aydin et al., 2022). Thus, residues of both parent veterinary and pharmaceutical compounds and their degradation products have been detected in soils (Biel-Maeso et al., 2018; Conde-Cid et al., 2018; Nuñez-Delgado et al., 2019; Mejías et al., 2021). These findings are being taken into account by the regulatory bodies of the European Union, leading in the last two decades to increased investigations concerning the presence of these emergent contaminants such as antimicrobial compounds on soil-plant ecosystems (Caracciolo et al., 2015; Tasho and Cho, 2016; Pan and Chu, 2017; Madikizela et al., 2018; Gworek et al., 2021; Bolesta et al., 2022; Yin et al., 2023).
Soil microorganisms play a major role in soil quality and long-term sustainability of agricultural terrestrial ecosystems since they control the breakdown of organic matter and hence the net fluxes of carbon and nutrients through the decomposition, mineralization, and immobilization processes (Pankhurst et al., 1996; Nannipieri et al., 2003). However, most studies concerning the impact of emergent contaminants such as antibiotics on terrestrial ecosystems are focused on their presence, behaviour, and risk assessment on human health and aquatic systems (Li, 2014; Bastos et al., 2020; Aydin et al., 2022). It is well known that antimicrobials for veterinary and human medicine can drastically modify non-target organisms living in the agricultural soils and hence alter biodiversity and ecosystem functions. Surprisingly, despite interest in the topic, the investigations addressing the impact of antibiotics on autochthonous microorganisms in the terrestrial ecosystems are scarce. The investigations concerning the impact of different groups of pharmaceutical compounds on microbial communities of agricultural soils located through the world were collected in a recent review by Cycón et al. (2019). The review clearly evidences that the response of microorganisms to the presence of these compounds measured by means of different parameters related to their mass, activity, and diversity (microbial C, total PLFA biomass and specific microbial groups PLFA biomass, soil respiration, bacterial growth, soil enzymes, microbial community structure), were variable; thus, although a negative temporal effect was often observed on microorganisms living in the soil, non effect, or even a positive effect, could also be detected. This is in agreement with recent investigations conducted by our research group performed with acid agricultural soils located in Galicia (NW Iberian Peninsula) added with antibiotics of different groups (tetracyclines, sulfonamides, fluorquinones, penicillins, cephalosphorine, diaminopyridine, β-lactams) (Santás-Miguel et al., 2020a; Santás-Miguel et al., 2020b; Santás-Miguel et al., 2020c; Rodríguez-González et al., 2021; Santás-Miguel et al., 2021; Rodríguez-González et al., 2022; Santás-Miguel et al., 2022; Rodríguez-González et al., 2023).
The World Health Organization (2017) considers the macrolides to be critical antibimicrobials of the highest priority, besides being the second most commonly used group of antibiotics in Europe (European Centre for Disease Prevention and Control, 2018). Clarithromycin is one of the most frequently prescribed macrolides in human medicine, therefore environmental risk investigations about the presence of this compound in soils are necessary (McLaughlin and Belknap, 2008; Baumann et al., 2015; Senta et al., 2019; Aydin et al., 2022). Recently, we examined the bacterial growth of 12 agricultural soils, both with no additions and with the addition of increasing concentrations of clarithromycin over time (1, 8, and 42 incubation days) (Rodríguez-González et al., 2021). In general, the data showed an initial inhibitory (toxic) effect which disappeared over time; however, in some cases, surprisingly, bacterial growth rates reached values higher than those in the corresponding unpolluted soil after 42 days of incubation. The aim of this laboratory experiment is to determine whether these bacterial growth changes were accompanied by changes in biomass and microbial community structure.
Material and Methods
Soils
The study was conducted with four agricultural soils located in the temperate humid zone (Galicia, NW Iberian Peninsula) which have not been previously treated with antibiotics. For each soil, 15–20 subsamples, collected randomly from the top 20 cm of the A horizon, were mixed, sieved (<2 mm), thoroughly homogenized, and air-dried. The main soil properties, which have been previously determined by Rodríguez-González et al. (2022), are shown in Table 1. They showed different texture, similar pH (ranges of pH in water 5.1–5.6), and different organic matter content (1.61%–6.8% C).
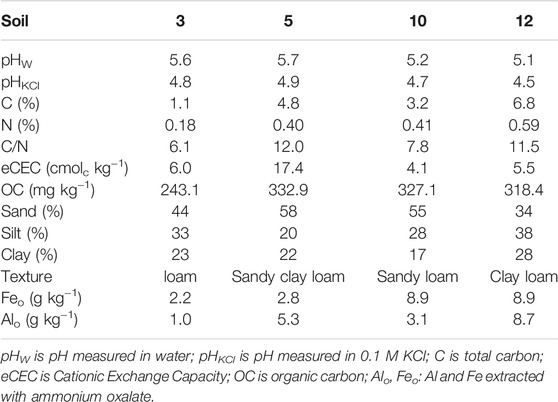
TABLE 1. Main properties of the four acid soils studied (adapted from Rodríguez-González et al., 2022).
Experimental Set Up
Of each of the four air-dried soil samples, 100 g was moistened to up to 70% of the water holding capacity and incubated in the dark at 22°C for 15 days to recover and achieve the stabilization of bacterial growth (Meisner et al., 2013). After that, they were separated into 8 centrifuge tubes (12 g in each tube) to be spiked with 7 different concentrations of clarithromycin (0.49, 1.95, 7.81, 31.25, 125, 500, and 2,000 mg kg−1 of soil), using talc as carrier to facilitate the mixture of the antibiotic with the soil and a blank to which the same amount of talc (48 mg) was added, but without antibiotic. These concentrations allowed the study of the effects of the antibiotic in a range that encompasses the toxicity of the antibiotic on soil bacteria from blank (no toxicity) to levels that suppress bacterial growth at high levels (Rodriguez-Gonzalez et al., 2021). The different mixtures of soil and antibiotic were incubated under controlled conditions (22°C, 70% of water holding capacity, darkness). After 8 days of incubation, 2 g samples were extracted in triplicate from each mixture and PLFA analysis was made. The same process was repeated after 42 days. Sampling was destructive for each treatment-time combination, resulting in a total of 192 microcosms (4 soils × 8 concentrations × 3 replicates × 2 times). The PLFA estimates were made using a representative composite soil sample of each antibiotic treatment obtained by mixing the three incubation replicates.
Phospholipid Fatty Acids (PLFA) Analysis
The total biomass (TotalPLFA) and the biomass of the specific microbial groups as well as the microbial community structure were estimated using the procedure and the nomenclature described by Frostegård et al. (1993). A detailed description of the method is given by Rodríguez-González et al. (2022). TotalPLFA was estimated as the sum of all the extracted PLFAs. The following PLFAs fatty acids were used as indicators of biomass of specific microbial groups: bacterial biomass (BactPLFA), the sum of i15:0, a15:0, 15:0, i16:0, 16:1ω9, 16:1ω7t, i17:1ω8, i17:0, a17:0, 17:0, cy17:0, 18:1ω7, and cy19:0 PLFAs; fungal biomass (FungPLFA), 18:2ω6 PLFA; actinobacteria biomass (ActPLFA), the sum of 10Me16:0, 10Me17:0, and 10Me18:0 PLFAs; Gram-positive bacteria biomass (G+PLFA), the sum of i14:0, i15:0, i16:0, a15:0, i17:0, and a17:0 PLFAs; and Gram-negative bacteria biomas (G−PLFA), the sum of cy17:0, cy19:0, 16:1ω7c, and 18:1ω7 PLFAs (Santás-Miguel et al., 2020b). In order to detect environmental effects (soil, incubation, antibiotic addition) on the soil microbial community structure, the concentrations of all the individual PLFAs, expressed in mole percent and logarithmically transformed, were subjected to principal component analysis (PCA). All statistical analyses were made using the SPSS 15.0 statistical package.
Results and Discussion
The values of total (TotalPLFA) and specific microbial biomass (FungPLFA, BactPLFA, G−PLFA, G+PLFA, and ActPLFA) obtained for soil groups with nothing added and with different doses of the antibiotic clarithromycin added after 8 and 42 days of its application are shown in Figures 1, 2, respectively. In general, the values observed for the two sampling times were quite similar. Therefore, for each soil type, the data obtained for un-treated and treated samples after 8 and 42 days of incubation were grouped together. Furthermore, in order to facilitate the interpretation of the results, the mean values of soil samples added with the 7 doses of clarithromycin were compared with the corresponding soil without antibiotic addition. In control S3 soil, total biomass showed values (mean values ±SE) of 35 ± 6 nmol g−1 and FungPLFA, BactPLFA, G−PLFA, G+PLFA, and ActPLFA values of 0.47 ± 0.16, 17.8 ± 3.1, 6.6 ± 0.8, 9.9 ± 2.1, and 2.7 ± 0.5 nmol g−1, respectively. Similar values were found in the samples treated with clarithromycin, in which TotalPLFA, FungPLFA, BactPLFA, G−PLFA, G+PLFA, and ActPLFA values were 37 ± 3, 0.52 ± 0.07, 18.4 ± 1.1, 7 ± 0.5, 10.2 ± 0.5, and 2.9 ± 0.6 nmol g−1, respectively. In S5, S10, and S12 soils, the magnitude levels of the total and specific biomass values were quite similar and much higher than those observed for S3 soil (Figures 1, 2). These results can be explained by the organic C content of the soils, which is found to be positively correlated with the biomass estimates (Díaz-Raviña et al., 1988; Díaz-Raviña et al., 1993; Leirós et al., 2000). In general, as a consequence of antibiotic addition, the values of total and specific biomass of the S5, S10, and S12 soils increased. Thus, TotalPLFA biomass in these soils ranged from 117 ± 20 and 168 ± 33 nmol g−1 in control samples and from 162 ± 30 and 224 ± 56 nmol g−1 in antibiotic treated samples. FungPLFA biomass showed the lowest values of the specific microbial groups, ranging from 1.31 ± 0.32 and 1.88 ± 0.56 nmol g−1 in control soils and from 1.49 ± 0.47 and 4.16 ± 3.5 nmol g−1 in the soils added with clarithromycin. In contrast, BactPLFA biomass showed the highest values of the specific groups ranging from 49.3 ± 8.6 and 80.8 ± 18.5 nmol g−1 in un-treated control soils, and from 70.3 ± 26.4 and 104.2 ± 24.8 nmol g−1 in antibiotic polluted soils. G−PLFA biomass values varied from 16 ± 2.3 and 31.2 ± 10.2 nmol g−1 in control soils and from 26.7 ± 14 and 35 ± 7.9 nmol g−1 in treated soils, while G+PLFA biomass ranged from 29.3 ± 5.8 and 40.3 ± 9.7 nmol g−1 in control soils and from 41.2 ± 11.5 and 64.15 ± 15.33 nmol g−1 in antibiotic polluted soils. Finally, ActPLFA biomass ranged from 10.9 ± 1.9 and 14.9 ± 3.2 nmol g−1 in un-treated control soils and from 14 ± 4.6 and 20 ± 5.1 nmol g−1 in antibiotic polluted soils.
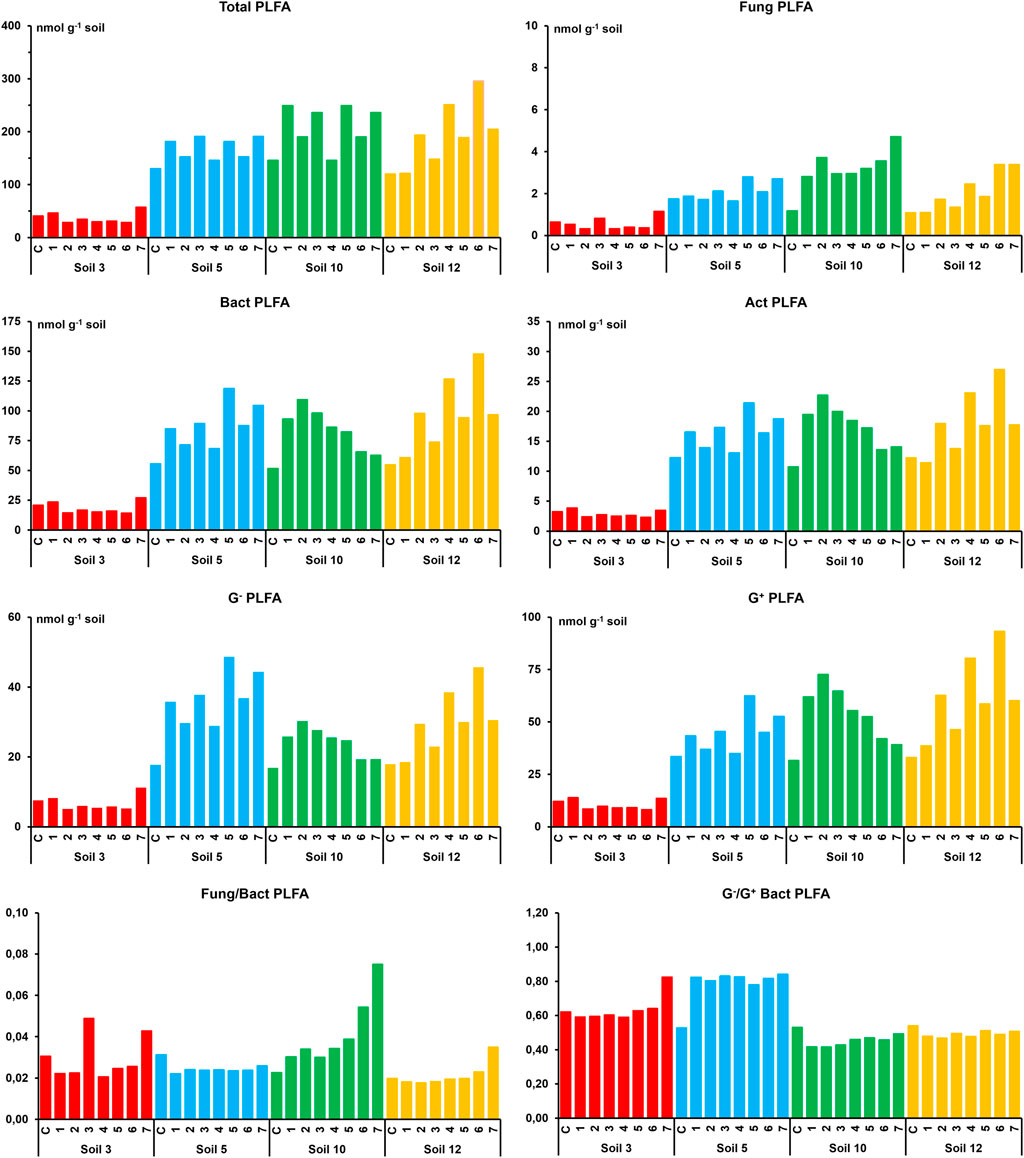
FIGURE 1. Total and specific microbial biomass values (fungal, bacterial, actinobacteria, Gram-negative bacteria and Gram-positive bacterial) in four studied soils with nothing added (C) and with the different doses of clarithromycin after 8 days of incubation. Doses: 1 (0.49), 2 (1.95), 3 (7.81), 4 (31.25), (5) 125, 6 (500), and 7 (2000) mg kg−1 of soil.
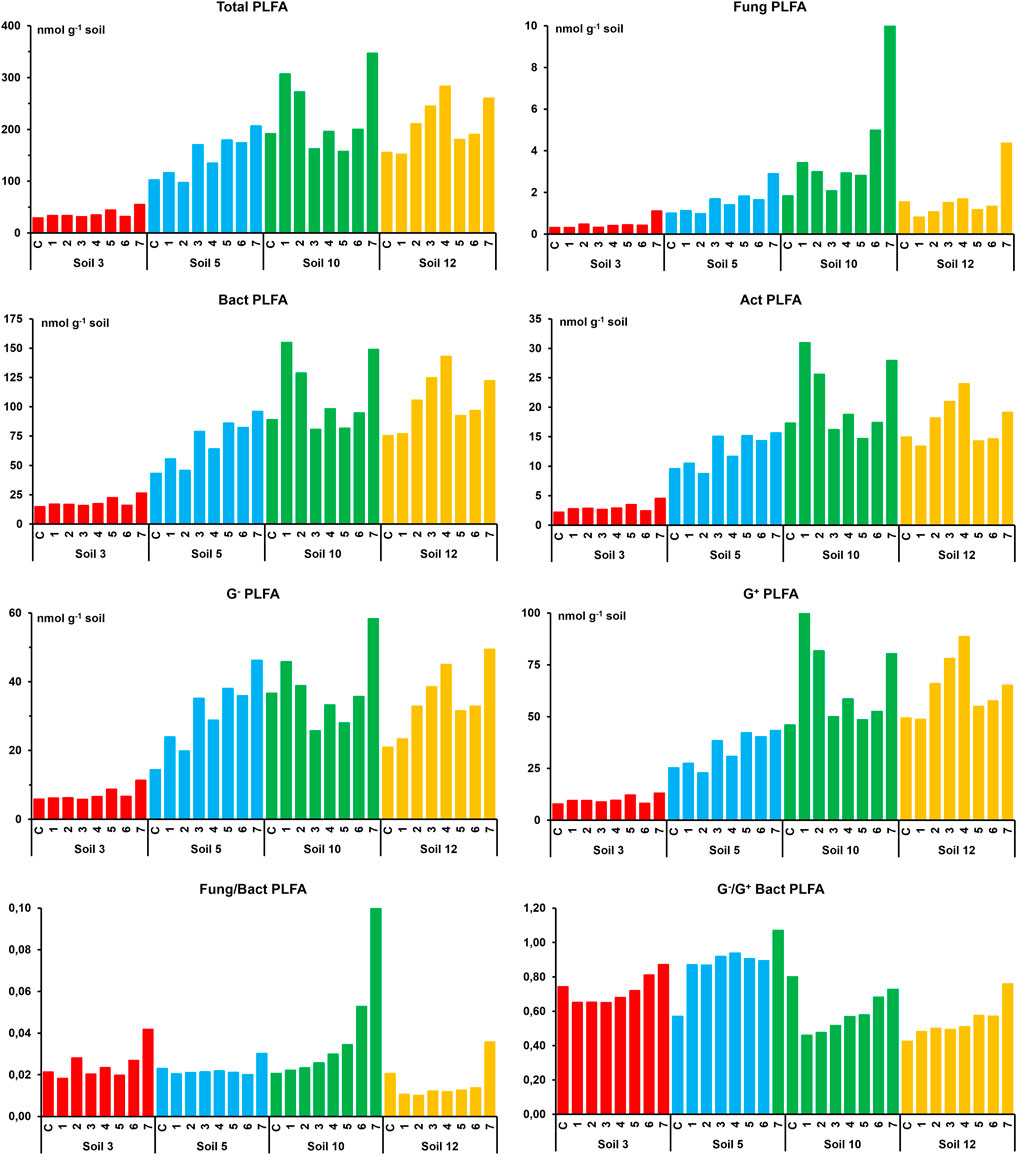
FIGURE 2. Total and specific microbial biomass values (fungal, bacterial, actinobacteria, Gram-negative bacteria, and Gram-positive bacterial) in four studied soils with nothing added (C) and with the different doses of clarithromycin after 42 days of incubation. Doses: 1 (0.49), 2 (1.95), 3 (7.81), 4 (31.25), (5) 125, 6 (500), and 7 (2000) mg kg−1 of soil.
The total and specific biomass PLFA values in both the control studied soils and the corresponding soils added with clarithromycin, were within the reported range given for other soils of the same area added with other organic compounds such as atrazine (Mahía et al., 2011), tetracyclines, cyproflaxin, trimethoprim, and amoxicillin (Santás-Miguel et al., 2021; Rodríguez-González et al., 2022). Likewise, the relative importance of different specific groups (FungPLFA, BactPLFA, G−PLFA, G+PLFA, and ActPLFA) with respect to the TotalPLFA, as well as the ratios of Fung/Bact PLFA and Gr−/G+ PLFA, were similar to those reported in the mentioned studies. In agreement with a previous study, the lowest total and specific biomass values were exhibited by the soil with the lowest organic matter content (Rodríguez-González et al., 2022).
In four studied soils, after the incubation of the soils added with clarithromycin, the values of the total and specific biomass were generally similar (S3 soil) or higher (soils S5, S10, and S12 soils) than those in the equivalent control soils. Consequently, a positive effect of antibiotic addition, with increases up to 2 times the control value, was detected at medium- (8 incubation days) and long-term (42 days). Comparison of four soil trends at two sampling times showed slight differences among them, with increases slightly higher at 42 days for S3 and S5 soils while the opposite was observed for S10 and S12 soils. The higher biomass values, which were dose dependent, showed that fungal and Gram− bacterial groups are more favoured by the antibiotic addition than the bacterial and Gram+ bacterial groups. This trend was evidenced by the values of the Fung/Bact PLFA and Gram−/G+ PLFA, which is coincident with findings of other studies on this topic (Hammesfahr et al., 2008; Unger et al., 2012; Chen et al., 2013; Rodríguez-González et al., 2022). Inconsistent results were reported in the literature concerning the impact of veterinary and human antibiotics on biomass of soil microbial communities estimated by the PLFA method. According to the results of the present investigation, some authors showed that microbial biomass increased (Cordova-Kreylos and Scow, 2007) while other researchers observed that PLFA biomass values were unaffected or decreased as a consequence of the antibiotic presence (Thiele-Bruhn and Beck, 2005; Cui et al., 2014). The studies also showed that this variable response of microbial communities to the antibiotic addition depended on soil characteristics, type, and dose of antibiotic as well as time passed after its application (Cycón et al., 2019; Santás et al., 2020b; Santás et al., 2021; Rodríguez-González et al., 2022).
Figure 3 shows the results of the principal component analysis performed with the whole PLFA data set obtained for the four studied soils (S3, S5, S10, S12) with nothing added (control soil) and with different concentrations of clarithromycin added (0.49, 1.95, 7.81, 31.25, 125, 500 and 2,000 mg kg−1 of soil) after 8 and 42 days of incubation. The plane defined by the first and second component, accounting for the 43% of the variation, separated the soils independently of antibiotic addition and the time passed after its application. Samples of the same soil at two sampling times (8 and 42 days) and the antibiotic addition were grouped together and separated from the rest of the soils. S10 samples, having higher concentrations of i16:0, a17:0, 10Me18:0 and 10Me17:0 PLFAs, were located in the positive part of axis 1, S12 and S3 samples in the middle part and, finally, S5 samples, having higher concentrations of PLFAs 18:0, 16:0, cy19:0, 16:1ω9, and 18:1ω7, in the negative part. The axis 2 clearly separated S3 samples, having higher concentrations of PLFAs, at 17:0, 19:1a, 18:2ω6, 18:1, and 18:1ω9, from the rest of the soils (S5, S10, and S12), with higher values of 10Me16:0, 16:1ω5, i14:0, and i15:0. This axis also differentiated samples showing higher antibiotic concentrations, located in its negative part, which exhibited higher concentrations of PLFAs 18:2ω6 and 18:1ω9, indicative of fungi, and PLFA 19:1a, characteristic of G− bacteria. Likewise, for each soil, a slight separation of samples incubated for 8 and 42 days was observed along axis 1 as well as a more defined separation of samples according the antibiotic addition, particularly after 42 days of incubation at higher doses.
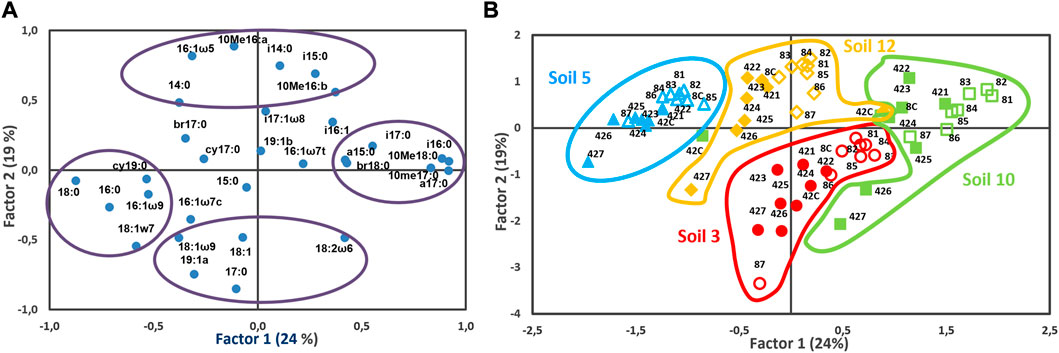
FIGURE 3. Principal component analysis ((A), variables; (B), samples) performed with the PFLA data set for the four studied soils with nothing added (C) and with the different doses of clarithromycin after 8 (open symbols) and 42 (filled symbols) days of incubation. Doses: 1 (0.49), 2 (1.95), 3 (7.81), 4 (31.25), (5) 125, 6 (500), and 7 (2000) mg kg−1 of soil.
To properly analyze the influence of the antibiotic addition on microbial community structure without the masking effect of time passed after its application, principal component analysis was made for each time separately (8 and 42 days after incubation) (Figure 4). Similar results were observed independently of sampling time (8 and 42 days of incubation). Again, the plane was defined by axis 1 and 2, accounting for 46% of the variation, samples of same soil were grouped together and separated from the other soils. Along axis 1, accounting for 23% of the variation, samples of S3 and S10 were located in the positive part of axis 1, while samples of S5 and S12 were situated in the negative part. The axis 2, accounting for 23% of the variation, clearly distinguished between samples with nothing added and those with an increasing dose of clarithromycin. Thus, in general, for all soils, independently of soil and sampling time, the highest effect of clarithromycin was observed at higher doses. It should be noted that, for all soils, the samples with higher doses of clarithromycin antibiotic had a relatively higher abundance of fungi, as indicated by PLFAs 18:2ω6 and 18:1ω9, and G− bacteria, as indicated by PLFAs 18:1ω7, 19:1a, 16:1ω7c, and cy19:0.
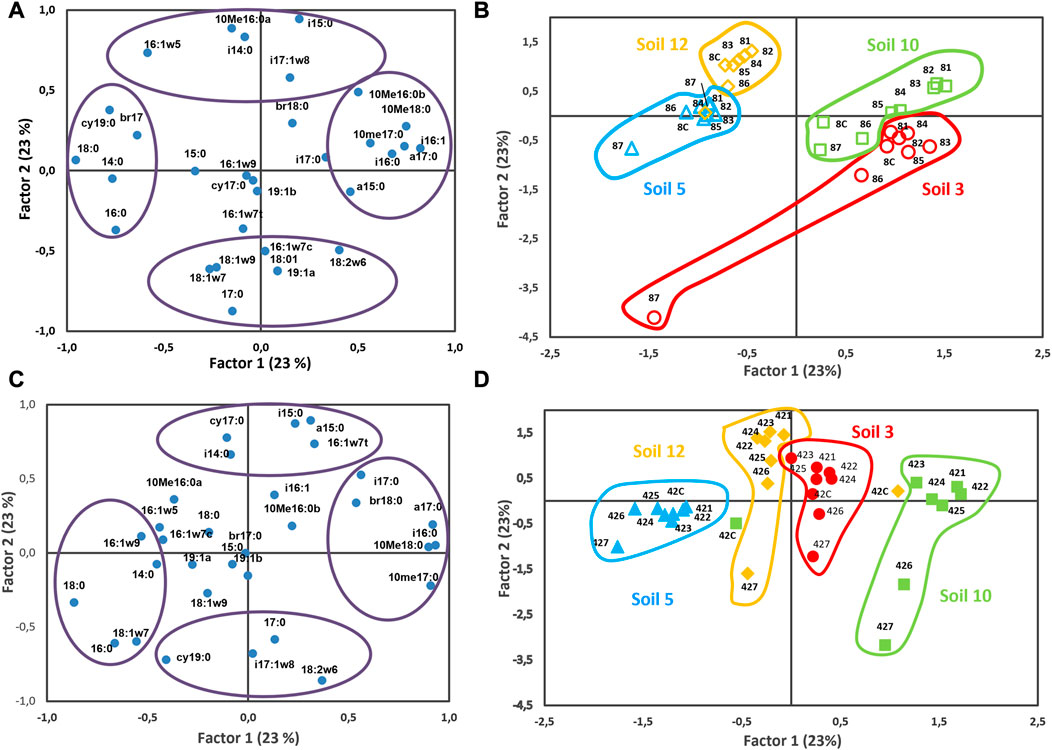
FIGURE 4. Principal component analysis performed with the PFLA data set for the four studied soils with nothing added (C) and with the different doses of clarithromycin after 8 (A,B) and 42 (C,D) days of incubation. Doses: 1 (0.49), 2 (1.95), 3 (7.81), 4 (31.25), (5) 125, 6 (500), and 7 (2000) mg kg−1 of soil.
Therefore, our data clearly demostrated that the main factor determining the source of variation of PLFA profiles among samples are soil characteristics followed by the incubation time and, to a lesser extent, the antibiotic dose. This is consistent with numerous field and laboratory studies concerning phospholipid fatty acid profiles performed with different soils following the impact of numerous stress agents (e.g., wildfire, prescribed fire, soil heating, fire retardants, post-fire rehabilitation treatments, vegetation type, soil management, climatic conditions, herbicide application, heavy metal pollution) which found that microbial communities of the same soil with different disturbances were grouped together and separated from microbial communities of other soils (Bossio et al., 1998; Díaz-Raviña et al., 2006, 2018; Fernández-Calviño et al., 2010; Mahía et al., 2011; Barreiro et al., 2015; Lombao et al., 2015). Likewise, a similar medium-term response of microbial communities was observed following the addition of antibiotics of both veterinary use such as tetracyclines (Santás-Miguel et al., 2021) and human use such as ciprofloxacin, trimethoprim, and amoxicillin (Rodríguez-González et al., 2022) to some soils of the same temperate humid zone.
The results of total and specific microbial biomass and microbial community structure determined by means of PLFA analysis were somehow coincident, showing that: a) the clarithromycin addition favoured fungi and G− bacteria more than bacteria and G+ bacterial and b) the effects observed short-term (8 days of incubation) changed very little at medium-term (42 days of indication). The PLFA pattern distinguished different microbial communities following the application of increasing concentrations of clarithromycin; however, this analysis does not allow us to determine if its impact is positive or negative. This information is derived from total and specific microbial biomass data which showed a positive influence on soil microbial communities. The results of a study of Díaz-Raviña et al. (1988) concerning the soil respiration and mineralization of soil organic matter (SOM) in soils with nothing added and with glucose added, located in the temperate humid zone, demonstrated that the C availability is the limiting factor of microbial community activity. Thus, the positive effect of clarithromycin on soil microorganisms can be explained by the use of the antibiotic as a source of C (Liao et al., 2016; Schofield, 2018) and/or the increase of C and nutrient availability derived from the death of non-target bacteria (bactericide effect) (Díaz-Raviña and Bååth, 1996; Rajapaksha et al., 2004). Likewise, an increased mineralization of the native SOM (priming effect) caused by trace concentrations of “tigger solutions” of original chlaritromycin and its secondary metabolites can not be discarded. The magnitude and the duration (8 and 42 days) of the increases of the microbial biomass seem to support the latter hypothesis. Our results are also in agreement with findings of other authors concerning the respiration or bacterial growth rate values following the addition of glucose, amino acids, root exudates (De Nobili et al., 2001), herbicides (Mahía et al., 2008), and antibiotics of veterinary or human origin (Rodríguez-González et al., 2022) to different soils. It should be noted that an increased biomass and bacterial growth rates cannot be necessarily related to an increase in the soil quality status, particularly in these acid soils with low C and nutrient availability where soil quality is closely related to quantity and quality of soil organic matter (SOM) (Carballas et al., 2015). Thus, for example, in soil S3 exhibiting the lowest SOM content and C/N ratio, an increased SOM over a prolonged time (more than 42 days) can provoke a decrease in SOM content and hence also in soil quality.
The values reported in the literature for half-lifes of clarithromycin in soils, estimated in laboratory experiments using labelled compounds, ranged from 8 days to almost no dissipation (Kodešová et al., 2016; Topp et al., 2016). This behavior can be explained by the characteristics and concentration of the clarithromycin, the soil properties, as well as the time passed after application. In general, the higher half-life values or long-term persistence of clarithromycin were observed in more polluted soils with high sorption capacity (Cycón et al., 2019). This is associated not only with clarithromycin affinity by soil and chemical properties such as pH and properties related to the effective cation exchange properties (Rodríguez-González et al., 2022), but also with the soil microbial properties. The latter hypothesis is confirmed by Kodešová et al. (2016) who found higher dissipation half-lives in soils of better quality and, hence, with better microbial conditions for biodegradation processes than in lower quality soils. The history of field exposure to clarithromycin also showed a great influence on the response of microbial communities to the recent application of this antibiotic to the same soil; thus, repeated annual exposure to the antibiotic provided selective pressure to provoke changes in microbial composition (increase of tolerant microorganisms and decrease of sensitive ones) and, hence, a faster dissipation of clarithromycin (Topp et al., 2016; Lau et al., 2020). Evidence for long-term exposure leading to accelerated biodegradation of organic compounds in soils was previously detected for herbicides (Barriuso and Houot, 1996; Mahía et al., 2011).
In a previous investigation, we examined the bacterial growth rates of soils S3, S5, S10, and S12 not added and added with the same increasing concentration of clarithromycin over time (1, 8, and 42 days) (Rodríguez-González et al., 2022); therefore, data can be properly compared with those obtained in this investigation. For all soils, an inhibitory effect of clarithromycin on bacterial growth was detected; however, this toxicity disappeared gradually with time (8 days) and was no longer found after 42 days. In fact, for soil S3 after 8 days and for all soils after 42 days of incubation, bacterial growth rates increased exponentially between 8 and 42 days at higher doses of the antibiotic. In the present work, a positive effect of antibiotic was shown on total and specific microbial biomass values after both 8 and 42 days, the amended soils reaching values 1.5–2 times higher than those in the corresponding unpolluted soil. Therefore, the data supported our initial hypothesis about the fact that changes in bacterial growth observed as a consequence of clarithromycin addition were accompanied by changes in both microbial community composition and total and specific microbial biomass.
Conclusion
In summary, the results of the present study clearly demonstrated residual effects on total and specific microbial biomass (shifts in the positive direction) in these agricultural acid soils as a consequence of the clarithromicyn addition after 42 days of application. Since these microbial PLFAbiomass changes are associated with shifts in the microbial composition (PLFA pattern), it is quite probable that clarithromycin can have also an impact on functional microbial diversity. Further investigations including the measurements of microbial parameters related to mass, activity, microbial community structure, and functional microbial diversity should be conducted in short-, medium- and long-term studies in order to gain a deeper understanding of the persistence period of clarythromycine in terrestrial ecosystems as well as its risk assessment in human health, which is associated with its potential negative impact on soil functioning.
Data Availability Statement
The raw data supporting the conclusion of this article will be made available by the authors, without undue reservation.
Author Contributions
Conceptualization; MA-E, DF-C, MD-R; Methodology: MA-E, DF-C, MD-R; Software: LR-G, VS-M, ÁM; Data curation: EG-C, ÁM, LR-G, MD-R; Writing-Original draft preparation: LR-G, VS-M, MD-R, ÁM; Visualization: MA-E, DF-C, MD-R, LR-G, VS-M; Investigation: MD-R, LR-G, VS-M, EG-C; Supervision: MA-E, DF-C, MD-R; Validation: MD-R, MA-E, DF-C; Writing- Reviewing and Editing: LR-G, MD-R, VS-M.
Funding
This study has been funded by the Spanish Ministry of Science, Innovation and Universities through the projects RTI2018-099574-B-C21 and RTI2018-099574-B-C22 (FEDER Funds). VS-M holds a postdoctoral fellowship (ED481B-2022-081) financed by Xunta de Galicia. LR-G hold a pre-doctoral fellowship (FPU21/04206) financed by Spanish Ministry of Universities. Funding for open access charge: Universidade de Vigo/CISUG.
Conflict of Interest
The authors declare that the research was conducted in the absence of any commercial or financial relationships that could be construed as a potential conflict of interest.
References
Aydin, S., Ulvi, A., Bedük, F., and Aydin, M. E. (2022). Pharmaceutical Residues in Digested Sewage Sludge: Occurrence, Seasonal Variation and Risk Assessment for Soil. Sci. Total Environ. 817, 152864. doi:10.1016/j.scitotenv.2021.152864
Barreiro, A., Fontúrbel, M. T., Lombao, A., Martín, A., Vega, J. A., Carballas, T., et al. (2015). Using Phospholipid Fatty Acid and Community Level Physiological Profiling Techniques to Characterize Soil Microbial Communities Following an Experimental Fire and Different Stabilization Treatments. Catena 135, 419–429. doi:10.1016/j.catena.2014.07.011
Barriuso, E., and Houot, S. (1996). Rapid Meneralization of the S-Triazine Ring of Atrazine in Soils in Relation to Soil Management. Soil Biol. Biochem. 28, 1341–1348. doi:10.1016/S0038-0717(96)00144-7
Bastos, M. C., Soubrand, M., Guet, T. L., Floch, É. L., Baudu, M., Casellas, M., et al. (2020). Occurrence, Fate and Environmental Risk Assessment of Pharmaceutical Compounds in Soils Amended with Organic Wastes. Geoderma 375, 114498. doi:10.1016/j.geoderma.2020.114498
Baumann, M., Weiss, K., Maletzki, D., Schüssler, W., Schudoma, D., Kopf, W., et al. (2015). Aquatic Toxicity of the Macrolide Antibiotic Clarithromycin and its Metabolites. Chemosphere 120, 192–198. doi:10.1016/j.chemosphere.2014.05.089
Biel-Maeso, M., Corada-Fernández, C., and Lara-Martín, P. A. (2018). Monitoring the Occurrence of Pharmaceuticals in Soils Irrigated with Reclaimed Wastewater. Environ. Pollut. 235, 312–321. doi:10.1016/j.envpol.2017.12.085
Bolesta, W., Głodniok, M., and Styszko, K. (2022). From Sewage Sludge to the Soil—Transfer of Pharmaceuticals: a Review. Int. J. Environ. Res. Public Health. 19, 10246. doi:10.3390/ijerph191610246
Bossio, D. A., Scow, K. M., Gunapala, N., and Graham, K. J. (1998). Determinants of Soil Microbial Communities: Effects of Agricultural Management, Season, and Soil Type on Phospholipid Fatty Acid Profiles. Microb. Ecol. 36, 1–12. doi:10.1007/s002489900087
Caracciolo, A. B., Topp, E., and Grenni, P. (2015). Pharmaceuticals in the Environment: Biodegradation and Effects on Natural Microbial Communities. A Rev. Pharm. Biomed. Anal. 106, 25–36. doi:10.1016/j.jpba.2014.11.040
Carballas, T., Rodríguez-Rastrero, M., Artieta, O., Gumuzzio, J., Díaz-Raviña, M., Martín, A., et al. (2015). “Soils of Temperate Humid Zone,” Editorial in The Soils of Spain (Switzerland: Springer), 49–144. Lancho, Coord.
Chen, W., Liu, W., Pan, N., Jiao, W., and Wang, M. (2013). Oxytetracycline on Functions and Structure of Soil Microbial Community. J. Soil. Sc. Plant Nutr. 13, 0–975. doi:10.4067/S0718-95162013005000076
Conde-Cid, M., Álvarez-Esmorís, C., Paradelo-Nuñez, R., Nóvoa-Muñoz, J. C., Arias-Estévez, M., Álvarez-Rodríguez, E., et al. (2018). Occurrence of Tetracyclines and Sulfonamides in Manures, Agricultural Soils and Crops from Different Areas in Galicia (NW Spain). J. Clean. Prod. 197, 491–500. doi:10.1016/j.jclepro.2018.06.217
Cordova-Kreylos, A. L., and Scow, K. M. (2007). Effects of Ciprofloxacin on Salt Marsh Sediment Microbial Communities. ISME J 1 (7), 585–595. doi:10.1038/ismej.2007.71
Cui, H., Wang, S. P., Fu, J., Zhou, Z. Q., Zhang, N., and Guo, L. (2014). Influence of Ciprofloxacin on Microbial Community Structure and Function in Soils. Biol. Fertil. Soils. 50 (6), 939–947. doi:10.1007/s00374-014-0914-y
Cycón, M., Mrozik, A., and Piotrowska-Seget, Z. (2019). Antibiotics in the Soil Environment—Degradation and Their Impact on Microbial Activity and Diversity. Front. Microbiol. 10, 338. doi:10.3389/fmicb.2019.00338
De Nobili, M., Contin, M., Mondini, C., and Brookes, P. C. (2001). Soil Microbial Biomass Is Triggered into Activity by Trace Amounts of Substrate. Soil Biol. biochem. 32, 1163–1170. doi:10.1016/S0038-0717(01)00020-7
Díaz-Raviña, M., and Bååth, E. (1996). Thymidine and Leucine Incorporation into Bacteria from Soils Experimentally Contaminated with Heavy Metals. Appl. Soil Ecol. 3, 225–234. doi:10.1016/0929-1393(95)00086-0
Díaz-Raviña, M., Carballas, T., and Acea, M. J. (1988). Microbial Biomass and Metabolic Activity in Four Acid Soils. Soil Biol. Biochem. 20, 817–823. doi:10.1016/0038-0717(88)90087-9
Díaz-Raviña, M., Acea, M. J., and Carballas, T. (1993). Microbial Biomass and its Contribution to Nutrient Concentrations in Forest Soils. Soil Biol. biochem. 25, 25–31. doi:10.1016/0038-0717(93)90237-6
Díaz-Raviña, M., Bååth, E., Martín, A., and Carballas, T. (2006). Microbial Community Structure in Forest Soils Treated with a Fire Retardant. Biol. Fertil. Soils 42 (6), 465–471. doi:10.1007/s00374-005-0036-7
Díaz-Raviña, M., Lombao, A., Barreiro, A., Martín, A., and Carballas, T. (2018). Medium-term Impact of Post-fire Emergency Rehabilitation Techniques on a Shrubland Ecosystems in Galicia (NW Spain). Span. J. Soil Sci. 8, 322–346.
European Centre for Disease Prevention and Control (ECDC) (2018). “Surveillance Report,” in Annual Epidemiological Report for 2016; Antimicrobial Consumption (Sweden: ECDC: Solna Municipality).
Fernández-Calviño, D., Martín, A., Arias-Estévez, M., Bååth, E., and Díaz-Raviña, M. (2010). Microbial Community Structure of Vineyard Soils with Different pH and Copper Content. Appl. Soil Ecol. 46, 276–282. doi:10.1016/j.apsoil.2010.08.001
Fijalkowski, K., Rorat, A., Grobelak, A., and Kacprzak, M. J. (2017). The Presence of Contaminations in Sewage Sludge. The Current Situation. J. Environ. Manag. 203, 1126–1136. doi:10.1016/j.jenvman.2017.05.068
Frostegård, A., Bååth, E., and Tunlip, A. (1993). Shifts in the Structure of Soil Microbial Communities in Limed Forests as Revealed by Phospholipid Fatty Acid Analysis. Soil Biol. Biochem. 25, 723–730. doi:10.1016/0038-0717(93)90113-P
Gworek, B., Kijeéwska, M., Wrzosek, J., and Graniewska, M. (2021). Pharmaceuticals in the Soil and Plant Environment: a Review. Water Air Soil Pollut. 232, 145. doi:10.1007/s11270-020-04954-8
Hammesfahr, U., Heuer, H., Manzke, B., Smalla, K., and Thiele-Bruhn, S. (2008). Impact of the Antibiotic Sulfadiazine and Pig Manure on the Microbial Community Structure in Agricultural Soils. Soil Biol. Biochem. 40 (7), 1583–1591. doi:10.1016/j.soilbio.2008.01.010
Kodešová, R., Kočárek, M., Klement, A., Golovko, O., Koba, O., Fér, M., et al. (2016). An Analysis of the Dissipation of Pharmaceuticals under Thirteen Different Soil Conditions. Sci. Total Environ. 544, 369–381. doi:10.1016/j.scitotenv.2015.11.085
Kümmerer, K. (2009). Antibiotics in the Aquatic Environment—A Review—Part I. Chemosphere 75, 417–434. doi:10.1016/j.chemosphere.2008.11.086
Lau, C. H. L., Tien, Y. C., Stedtfeld, R. D., and Topp, E. (2020). Impacts of Multi-Year Field Exposure of Agricultural Soil to Macrolide Antibiotics on the Abundance of Antibiotic Resistance Genes and Selected Mobile Genetic Elements. Sci. Total Environ. 727, 138520. doi:10.1016/j.scitotenv.2020.138520
Leirós, M. C., Trasar-Cepeda, C., Seoane, S., and Gil-Sotres, F. (2000). Biochemical Properties of Acid Soils under Climax Vegetation (Atlantic Oakwood) in an Area of the European Temperate–Humid Zone (Galicia, NW Spain): General Parameters. Soil Biol. Biochem. 32, 733–745. doi:10.1016/S0038-0717(99)00195-9
Li, W. C. (2014). Occurrence, Sources, and Fate of Pharmaceuticals in Aquatic Environment and Soil. Environ. Pollut. 187, 193–201. doi:10.1016/j.envpol.2014.01.015
Liao, X., Li, B., Zou, R., Dai, Y., Xie, S., and Yuan, B. (2016). Biodegradation of Antibiotic Ciprofloxacin: Pathways, Influential Factors, and Bacterial Community Structure. Environ. Sci. Pollut. Res. 23 (8), 7911–7918. doi:10.1007/s11356-016-6054-1
Lombao, A., Díaz-Raviña, M., Martín, M., Fontúrbel, M. T., Vega, J. A., Fernández, C., et al. (2015). Influence of Straw Mulch Application on the Properties of a Soil Affected by a Forest Wildfire. J. Soil Sci. 5, 26–40. doi:10.3232/SJSS.2015.V5.N1.03
Madikizela, L. M., Ncube, S. B., and Chimuka, L. (2018). Uptake of Pharmaceuticals by Plants Grown under Hydroponic Conditions and Natural Occurring Plant Species: A Review. Sci. Total Environ. 636, 477–486. doi:10.1016/j.scitotenv.2018.04.297
Mahía, J., Cabaneiro, A., Carballas, T., and Díaz-Raviña, M. (2008). Microbial Biomass and C Mineralization in Agricultural Soils as Affected by Atrazine Addition. Biol. Fertil. Soils 45, 99–105. doi:10.1007/s00374-008-0318-y
Mahía, J., González-Prieto, S. J., Martín, A., Bååth, E., and Díaz-Raviña, M. (2011). Biochemical Properties and Microbial Community Structure of Five Different Soils after Atrazine Addition. Biol. Fertil. Soils 47 (5), 577–589. doi:10.1007/s00374-011-0569-x
McLaughlin, A., and Belknap, A. (2008). Annual Kg Quantity of Medicinal Ingredients Distributed and Dispensedin Canada: Analysis if Intercontinental Medical Stadistics (IMS) Data for 2007. Excel Formal data. Environmental Impact Initiative, HPFB, Health Canada.
Meisner, A., Bååth, E., and Rousk, J., (2013). Microbial Growth Responses upon Rewetting Soil Dried for Four Days or One Year. Soil Biol. biochem. 66, 188–192. doi:10.1016/j.soilbio.2013.07.014
Mejías, C., Martín, J., Santos, J. L., Aparicio, I., and Alonso, E. (2021). Ocurrence of Pharmaceuthicals and Their Metabolites in Sewagw Sludge and Soil: a Review on Their Distribution and Environmental Risk Assessment. Trends Environ. Anal. Chem. 30, e00125. doi:10.1016/j.teac.2021.e00125
Nannipieri, P., Ascher, J., Ceccherini, M. T., Landi, L., Pietramellara, G., and Renella, G. (2003). Microbial Diversity and Soil Functions. Eur. J. Soil Sci. 54, 655–670. doi:10.1046/j.1351-0754.2003.0556.x
Nuñez-Delgado, A., Zhou, A., Necibi, C., Xu, Y., and Fernández-Calviño, D. (2019). Editorial of the VSI “Antibiotics and Heavy Metals in the Environment: Facing the Challenge”. Sci. Total Environ. 678, 30–32. doi:10.1016/j.scitotenv.2019.04.421
Pan, M., and Chu, L. M. (2017). Fate of Antibiotics in Soil and Their Uptake by Edible Crops. Sci. Total Environ. 599, 500–512. doi:10.1016/j.scitotenv.2017.04.214
Pankhurst, C. E., Ophel-Keller, K., Doube, B. M., and Gupta, V. V. S. R. (1996). Biodiversity of Soil Microbial Communities in Agricultural Systems. Biodivers. Conserv. 5, 197–209. doi:10.1007/BF00055830
Rajapaksha, R. M. C. P., Tobor-Kaplon, M. A., and Bååth, E. (2004). Metal Toxicity Affects Fungal and Bacterial Activities in Soil Differently. Appl. Environ. Microbiol. 70, 2966–2973. doi:10.1128/AEM.70.5.2966-2973.2004
Rodríguez-González, L., Santás-Miguel, V., Campillo-Cora, C., Arias-Estévez, M., and Fernández-Calviño, D. (2021). The Effect of Clarithromycin Toxicity on the Growth of Bacterial Communities in Agricultural Soils. Processes 9 (8), 1303. doi:10.3390/pr9081303
Rodríguez-González, L., Nuñez-Delgado, A., Álvarez-Rodríguez, E., García-Campos, E., Martín, A., Díaz-Raviña, M., et al. (2022). Effects of Ciprofloxacin, Trimethoprim, and Amoxicillin on Microbial Structure and Growth as Emerging Pollutants Reaching Crop Soils. Environ. Res. 214, 113916. doi:10.1016/j.envres.2022.113916
Rodríguez-González, L., Nuñez-Delgado, A., Álvarez-Rodríguez, E., Díaz-Raviña, M., Arias-Estévez, M., Fernández-Calviño, D., et al. (2023). Direct Toxicity of Six Antibiotics on Soil Bacterial Communities Affected by the Addition of Bio-Adsorbents. Environ. Pollut. 322, 121161. doi:10.1016/j.envpol.2023.121161
Santás-Miguel, V., Arias-Estévez, M., Díaz-Raviña, M., Fernández-Sanjurjo, M. J., Álvarez-Rodríguez, E., Núñez-Delgado, A., et al. (2020a). Effect of Oxytetracycline and Chlortetracycline on Bacterial Community Growth in Agricultural Soils. Agronomy 10 (7), 1011. doi:10.3390/agronomy10071011
Santás-Miguel, V., Díaz-Raviña, M., Martín, A., García-Campos, E., Barreiro, A., Núñez-Delgado, A., et al. (2020b). Medium-term Influence of Tetracyclines on Total and Specific Microbial Biomass in Cultivated Soils of Galicia (NW Spain). Span. J. Soil Sci. 10 (3), 217–232. doi:10.3232/SJSS.2020.V10.N3.05
Santás-Miguel, V., Rodríguez-González, L., Núñez-Delgado, A., Díaz-Raviña, M., Arias-Estévez, M., and Fernández-Calviño, D. (2020c). The Toxicity Exerted by the Antibiotic Sulfadiazine on the Growth of Soil Bacterial Communities May Increase over Time. Int. J. Environ. Res. Public Health 17 (23), 8773. doi:10.3390/ijerph17238773
Santás-Miguel, V., Díaz-Raviña, M., Martín, A., García-Campos, E., Barreiro, A., Núñez-Delgado, A., et al. (2021). Soil Enzymatic Activities and Microbial Community Structure in Soils Polluted with Tetracycline Antibiotics. Agronomy 11, 906. doi:10.3390/agronomy11050906
Santás-Miguel, V., Rodríguez-González, L., Núñez-Delgado, A., Álvarez-Rodríguez, E., Díaz-Raviña, M., Arias-Estévez, M., et al. (2022). Time-course Evolution of Bacterial Community Tolerance to Tetracycline Antibiotics in Agricultural Soils: A Laboratory Experiment. Chemosphere 291, 132758. doi:10.1016/j.chemosphere.2021.132758
Schofield, C. J. (2018). Antibiotics as Food for Bacteria. Nat. Microbiol. 3 (7), 752–753. doi:10.1038/s41564-018-0181-z
Senta, I., Kostanjevecki, P., Kritzman-Matasic, I., Terzic, S., and Ahel, M. (2019). Occurrence and Behavior of Macrolide Antibiotics in Municipal Wastewater Treatment: Possible Importance of Metabolites, Synthesis Byproducts, and Transformation Products. Environ. Sci. Technol. 53, 7463–7472. doi:10.1021/acs.est.9b01420
Tasho, R. P., and Cho, J. Y. (2016). Veterinary Antibiotics in Animal Waste, its Distribution in Soil and Uptake by Plants: A Review. Sci. Total Environ. 563, 366–376. doi:10.1016/j.scitotenv.2016.04.140
Thiele-Bruhn, S., and Beck, I. (2005). Effects of Sulfonamide and Tetracycline Antibiotics on Soil Microbial Activity and Microbial Biomass. Chemosphere 59, 457–465. doi:10.1016/j.chemosphere.2005.01.023
Thiele-Bruhn, S. (2003). Pharmaceutical Antibiotic Compounds in Soils – a Review. J. Plant Nutr. Soil Sci. 166, 145–167. doi:10.1002/jpln.200390023
Topp, E., Renaud, J., Surumah, M., and Sabourin, L. (2016). Reduced Persistence of the Macrolide Antibiotics Erythromycin, Clarithromycin and Azithromycin in Agricultural Soil Following Several Years of Exposure in the Field. Sci. Total Environ. 562, 136–144. doi:10.1016/j.scitotenv.2016.03.210
Unger, I. M., Goyne, K. W., Kennedy, A. C., Kremer, R. J., McLain, J. E. T., and Williams, C. F. (2012). Antibiotic Effects on Microbial Community Characteristics in Soils under Conservation Management Practices. Soil Sci. Soc. Am. J. 77, 100–112. doi:10.2136/sssaj2012.0099
World Health Organization (2017). “Critically Important Antimicrobials for Human Medicine 5th Revision 2016,” in Ranking of Medically Important Antimicrobials for Risk Management of Antimicrobial Tesistance Due to Non-human Use Uull (Geneva, Switzerland: World Health Organization).
Keywords: polluted soil, phospholipid fatty acids, persistent effects, antibiotics, microbial biomass
Citation: Rodríguez-González L, García-Campos E, Martín Á, Díaz-Raviña M, Arias-Estévez M, Fernández-Calviño D and Santás-Miguel V (2023) Microbial Communities as Affected by Clarithromycin Addition in Four Acid Soils (NW Iberian Peninsula). Span. J. Soil Sci. 13:11319. doi: 10.3389/sjss.2023.11319
Received: 28 February 2023; Accepted: 03 April 2023;
Published: 18 April 2023.
Edited by:
Avelino Núñez-Delgado, University of Santiago de Compostela, SpainCopyright © 2023 Rodríguez-González, García-Campos, Martín, Díaz-Raviña, Arias-Estévez, Fernández-Calviño and Santás-Miguel. This is an open-access article distributed under the terms of the Creative Commons Attribution License (CC BY). The use, distribution or reproduction in other forums is permitted, provided the original author(s) and the copyright owner(s) are credited and that the original publication in this journal is cited, in accordance with accepted academic practice. No use, distribution or reproduction is permitted which does not comply with these terms.
*Correspondence: Laura Rodríguez-González, bGF1cmEucm9kcmlndWV6LmdvbnphbGV6QHV2aWdvLmVz