- Instituto de Investigación en Agrobiotecnología (CIALE), Universidad de Salamanca, Salamanca, Spain
In recent decades, there has been increasing interest in studying the variability in soil water properties and, specifically, the spatiotemporal variability in the soil water content. This is motivated by the notable theoretical and applied research interests in soil moisture dynamics and their implications for many natural processes. This study aimed to study whether there are variations in the spatial pattern of the temporal stability of soil moisture over time and to analyze the possible influences of certain hydroclimatic (soil water content, precipitation, and evapotranspiration) and soil factors (texture, bulk density, and organic matter content) on these variations. This study was conducted within the Soil Moisture Measurement Stations Network of the University of Salamanca (REMEDHUS, Spain) under Mediterranean conditions, with daily surface moisture data (0–5 cm depth) obtained from 20 stations for the 2006-2023 period. The results showed differences between the average pattern obtained with the 18-year data series and that obtained with the data series for each year. In more than half of the years studied, the representative station differed from that derived from the average pattern. The mean annual precipitation and summer precipitation characteristics seem to be the main factors influencing the variability in the spatial pattern of the temporal stability of soil moisture.
Introduction
Soil moisture is a key status variable for understanding many hydrological processes that, in turn, are involved in a large variety of natural processes (geomorphological, climatic, ecological, etc.) that act at different spatio-temporal scales (Entin et al., 2000). Soil moisture is involved in the partitioning of net radiation into sensible and latent heat (Jones and Brunsell, 2009); it determines the amount of water available for evotranspiration (Kim et al., 2023), and it controls subsurface flow and the migration of chemicals towards aquifers (Su et al., 2022). Antecedent soil moisture is a key factor in hydrological and erosional modeling (Moragoda et al., 2022). Soil water content information is of special value for evaluating crop status (Sepulcre-Canto et al., 2012; Martínez-Fernández et al., 2016) and agricultural yield (Gaona et al., 2023). Knowledge of soil moisture processes and their spatial distribution provides essential information for climatic and hydrologic models (Martínez-Fernández et al., 2023). Finally, soil moisture is a good indicator of soil health and, therefore, for addressing such important issues as to achieving Sustainable Development Goals (Lal et al., 2021).
Interest in the study of the variability in soil water properties has persisted and has increased for several decades (Nielsen et al., 1973), as variability is a very relevant attribute from many perspectives (Singh et al., 2013; Castellini et al., 2019; Dari et al., 2019). Since the concept of the temporal stability of soil moisture was formalized in the 1980s (Vachaud et al., 1985) and understood as an expression of the temporal persistence of soil moisture, there has been much theoretical and applied research on this topic.
This interest was formalized, above all, from the assumption of the mean of the relative difference as a standard analysis metric for this parameter (Kachanoski and de Jong, 1988). Subsequently, many related works have been published from different perspectives (Martínez-Fernández and Ceballos, 2003; Wagner et al., 2008; Penna et al., 2013). This analysis has been applied in fields as diverse as hydrological modeling (Hao et al., 2020), remote sensing (Loew and Schlenz, 2011; Basu et al., 2024) and agriculture (Guber et al., 2008). Most of these works are based on studies with short or very short observation periods, from measurement campaigns (Gao et al., 2015; Dari et al., 2019; He et al., 2019) to multimonth series (Cosh et al., 2004; Burns et al., 2016) or a few years of measurements (Yee et al., 2016; Wang et al., 2017), at best.
Typically, in these studies, it is assumed that the resulting pattern of the temporal stability of soil moisture remains unchanged. Therefore, it is usually considered that those locations that characterize the wet or dry domain of an area always remain the same, that this pattern does not vary, and that the position considered representative of the average state of the entire spatial domain (Martínez-Fernández and Ceballos, 2005) does not change.
However, very few studies have focused on directly or indirectly analyzing the persistence of temporal stability patterns over time (Coopersmith et al., 2021). This could be related to the fact that the available series of soil moisture observations are short in many cases, in addition to the difficulty in establishing long-term measurement networks and databases. Fortunately, the situation is currently changing, and it is possible to identify cases (González-Zamora et al., 2019; Dorigo et al., 2021) in which the data series length allows us to address the persistence of temporal stability patterns.
The objectives of this work were to study whether there are variations in the spatial pattern of the temporal stability over time and to analyze the possible influences of certain hydroclimatic (soil water content, precipitation, and evapotranspiration) and soil factors (texture, bulk density, and organic matter) on the variations in spatiotemporal patterns. This approach was implemented using the databases of the Soil Moisture Measurement Station Network of the University of Salamanca (REMEDHUS) in Spain, with daily surface soil moisture (0–5 cm depth) series from 20 stations and an observation period of 18 years (2006–2023).
Materials and Methods
Study Area and Databases
This study was conducted using the databases of the REMEDHUS network (Figure 1) of the University of Salamanca (Spain). The REMEDHUS network covers an area of approximately 1,300 km2 in the central sector of the Duero Basin (41.1°-41.5°N and 5.1°-5.7°W) under semiarid Mediterranean climatic conditions and predominantly agricultural land use (Table 1). The main crops are rainfed cereals and, to a lesser extent, irrigated crops and vineyards. Scattered forestland and pasture areas are also present. This area is nearly flat (less than a 10% slope), and the altitude ranges from 700 to 900 m above sea level. The network comprises 20 automated soil moisture measurement stations (Table 1) equipped with Hydra probes (Stevens Water Monitoring System, Inc.) located at a depth of 5 cm, which record hourly soil moisture measurements. Hydra probe uses coaxial impedance dielectric reflectometry to provide accurate soil moisture data. The REMEDHUS network has been widely used in satellite soil moisture product validation studies and other applications (Sánchez et al., 2010; 2012; Sánchez-Ruiz et al., 2014; González-Zamora et al., 2015; 2016; 2019) and has been part of the International Soil Moisture Network (ISMN) (Dorigo et al., 2021) since its inception.
Average daily soil moisture data from each of the 20 stations during the period from 1 January 2006 to 31 December 2023 were used. In analyzing the influence of climatic variables, average daily precipitation and potential evapotranspiration (PET) data recorded at four automated meteorological stations (Figure 1) located in the REMEDHUS area were used. PET was calculated following the Penman–Monteith methodology (Allen et al., 1998).
Similarly, the network supports a database of soil characteristics (Ceballos et al., 2002; Gumuzzio et al., 2016) that were determined when the network was established, and correspond to each of the soil moisture measurement points. Sand, silt and clay content, bulk density and organic matter content data were obtained from this database (Table 1). These soil properties were assumed to be permanent, as the soil use of each soil moisture station did not change over the observation period. Even for a variable as organic matter of agricultural soils, there are studies that demonstrated that organic matter contents approached a steady-state at sites where agricultural management and crop rotation have remained relatively constant during decades (Gubler et al., 2019). These variables were used to analyze the role of soil properties in determining the variability in the temporal stability of soil moisture patterns.
Temporal Stability Analysis
The temporal stability of soil moisture pattern was determined via a parametric test of relative differences (Vachaud et al., 1985), which allows us to highlight differences, in terms of constancy in the temporal stability, between sampling points. The relative difference (δij) can be calculated as:
where
and
Sij is the moisture content at point i on day j, and N is the number of sampling points. Therefore, the average relative difference at each point can be defined as follows:
where m is the number of sampling days.
The standard deviation of the mean relative difference σ(δi) at each point can be calculated as an estimator of the temporal variability
As such, temporally stable measurement points can be defined as those with a low value of σ(δi).
Variability in the Temporal Stability Pattern Vs. Hydroclimatic and Soil Factors
To determine whether there were differences in the pattern of temporal stability of soil moisture over time, the mean relative difference (MRD) at each sampling point or soil moisture station was calculated for the full 18-year data series and for the individual data series of each year.
Moreover, the correlation coefficient R (Spearman) was calculated between the average pattern of the entire data series and that of the data series of each year. In this way, the mean and yearly patterns are more similar when R approaches one and vice versa. Such MRD and correlation analysis was also performed for each month instead of each year, with the goal of investigating whether the monthly patterns differed from the mean pattern.
With the aim of determining whether soil properties (permanent factors) influence the observed differences in temporal stability patterns, the MRD values of each year were correlated with those of the considered variables at each sampling point. Similarly, the R values resulting from this analysis were correlated with the values of the variables that define the hydroclimatic conditions (soil moisture, precipitation, and PET) in that area, both seasonally and annually. The aim was to study whether the influence of soil factors could be modulated by environmental conditions.
Similarly, it was studied whether hydroclimatic conditions (nonpermanent factors) influence the observed differences in temporal stability patterns, the R values resulting from the comparison of the annual MRD values with the average MRD values of the entire period were correlated with those of the considered variables (soil moisture, precipitation, and PET), both seasonally and annually.
Finally, with the goal of determining the general pattern that expresses the relationship between the soil moisture content and spatial variability, a comparative analysis was performed on a daily scale between the mean soil moisture and variance in the REMEDHUS network during the 18-year data period. This methodology has been widely employed (Owe et al., 1982; Famiglietti et al., 1998) to characterize this relationship.
Results and Discussion
The calculation of REMEDHUS MRD values for the entire series (2006–2023) revealed the average soil moisture stability pattern (Figure 2). In this pattern, the set of stations that represent the wet conditions of the network (positive values) and the set of stations that represent the dry state (negative values) could be observed. The first group included soils with a finer texture and a higher organic matter content (Table 1). In contrast, the soils in the second group exhibited a largely sandy texture. The determining roles of factors such as texture and organic matter in soil water behavior (Martínez-Fernández et al., 2021) and, specifically, in determining the temporal stability pattern of soil moisture have long been acknowledged (Martínez-Fernández and Ceballos, 2003; Vanderlinden et al., 2012). The results of MRD for the entire series oscillates between more than 150% (J12 station), on the wet side, and −77.5% (K4 station) on the dry side (Figure 2). This range of MRD is wider than the ones obtained in other studies under Mediterranean conditions (Gómez-Plaza et al., 2000; Brocca et al., 2012), but in the present study the textural range is wider, and the period of observations is much longer.
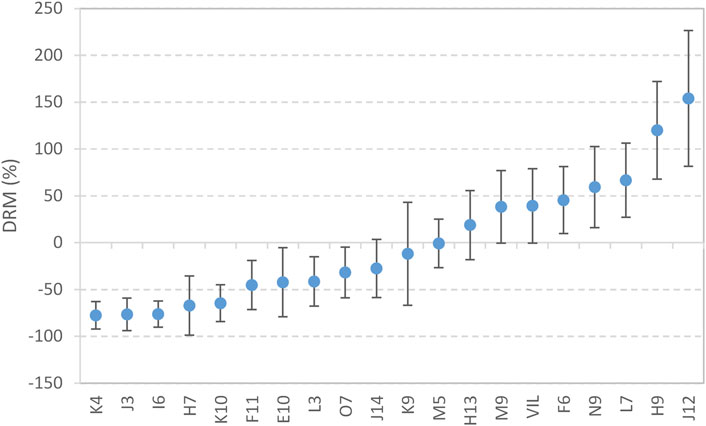
Figure 2. Mean relative differences (MRD) for each REMEDHUS measurement station for the 2006-2023 period. The bars indicate the standard deviation.
When comparing the pattern of the entire series with that of each year (Figure 3), it was observed that although the R values were almost always higher than 0.9, there were differences between some years. As an example, one can observe the case of the year with the lowest R value (2007). During that year, the stations of the dry side had a mean MRD of −53.4%, and 76.5% for those of the wet side, whereas the means for the entire series were −46.9% and 67.7%, respectively. Additionally, in 2007 the MRD of three stations (E10, H13 and K9) changed their sign regarding the mean pattern.
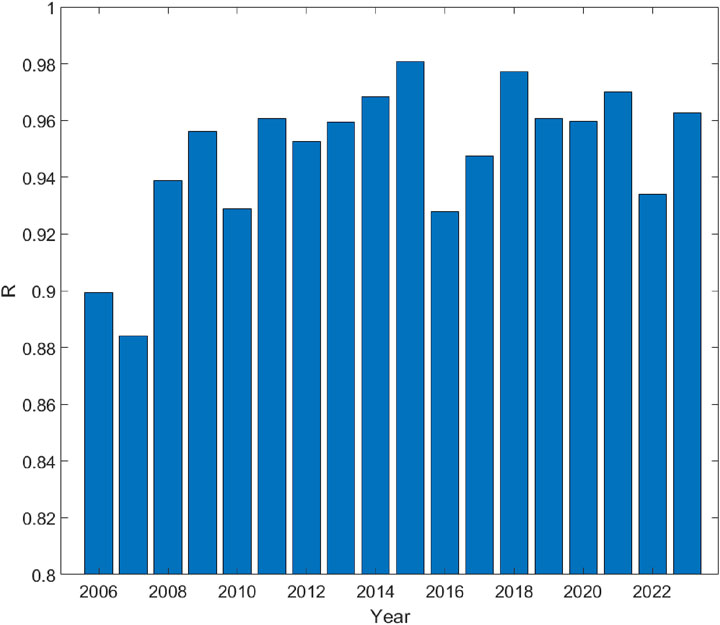
Figure 3. Spearman’s correlation coefficient between the mean relative difference (MRD) values obtained for the entire period (2006–2023) and those obtained for each year in the series.
Furthermore, the station representing the average state of the entire spatial domain (Martínez-Fernández and Ceballos, 2005), i.e., with an MRD closest to zero, varied from 1 year to another. According to the pattern of the complete series (Figure 2), this station was M5, but after analyzing the annual patterns, station M5 was identified as representing the average value of the network in only 8 of the 18 measurement years. However, this does not necessarily suggest that M5 was not a suitable representative sampling point for the entire observation period. But it is also relevant to point out that, although it is the station that shows the MRD value closest to zero in most years, this does not occur even in 50% of the years. Martínez-Fernández and Ceballos (2005) determined that approximately 1 year of measurements was required to determine the representative point of the average soil moisture under the environmental conditions of REMEDHUS. The results of this study indicated that this attribute could change annually.
Throughout the 18-year observation period, there were measurement stations with soil moisture status changes in some years. At some points, three stations changed from wet to dry (H13, M9, and VIL), with four stations changing from dry to wet (E10, J14, K9, and M5). Obviously, the MRD values at these stations were close to zero. For this reason, M5, although representative of the average soil water content in the area, also exhibited the most state changes.
The monthly-scale correlation analysis results (Figure 4A) showed hardly any differences between the different months, and no pattern could be observed throughout the year. However, when the average values of the standard deviation of each month were analyzed (Figure 4B), there were clear differences between the different months. The standard deviation of the MRD indicated a yearly pattern that was the opposite to that typically observed for the aridity index (AI) under Mediterranean conditions (Salvati et al., 2015). Arid months (AI > 0.2), which are summer months, exhibited the highest standard deviation values. Thus, the sampling points remained less temporally stable in summer.
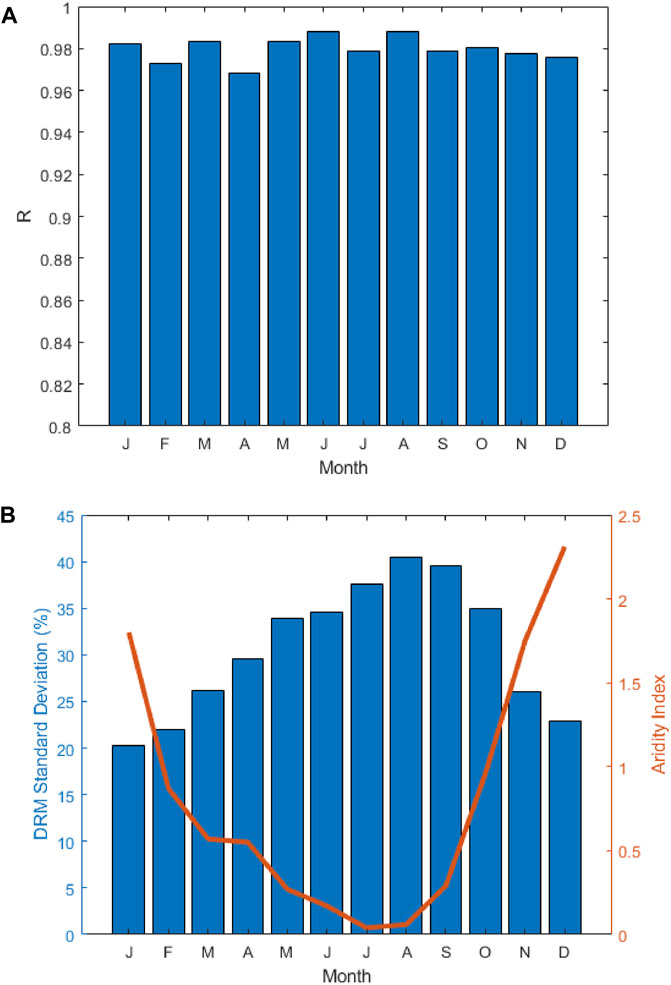
Figure 4. Spearman’s correlation coefficient between the average mean relative differences (MRD) pattern and that corresponding to each month of the year (A). Distribution of the monthly mean standard deviation of the MRD (B).
In a study using seven soil moisture measurement networks in the U.S., Coopersmith et al. (2021) analyzed temporal stability patterns from year to year and correlated them with the average pattern. Although they found that the general pattern was maintained overall, they observed differences, as the number of stations representative of wet and dry states also varied from year to year. The correlation coefficient of the comparison in their study was, in most cases, greater than 0.85, with notable variability, similar to the observations in the present study (Figure 3). However, their results can hardly be compared with those of the present study because, in their work, neither the same number nor the same stations were used every year in their data series.
With the aim of investigating the factors driving the variability depicted in Figure 3, the MRD values for each year and each measuring station were correlated with the associated soil factors (Figure 5). Although slight variations were observed over time, the leading role of texture and organic matter was maintained annually. With the aim of studying whether these variations could be related to hydroclimatic conditions, the R values were correlated with the mean values of precipitation, PET and soil moisture at the annual and seasonal scales. The analysis results did not reveal significant R values at either the annual or seasonal scale (Tables 2, 3, respectively). Although no R value was significant, the highest R values at the annual scale were observed for precipitation, and the highest R values at the seasonal scale were obtained in summer. Moreover, the relationship between precipitation and sand content occurred very close to the significance threshold (p < 0.05). Although these results were inconclusive, they indicated that summer precipitation may impose an accentuating effect on the already decisive role fulfilled by textural fractions in the study area, as was observed in previous works (Ceballos et al., 2002; Gumuzzio et al., 2016; Martínez-Fernández et al., 2021).
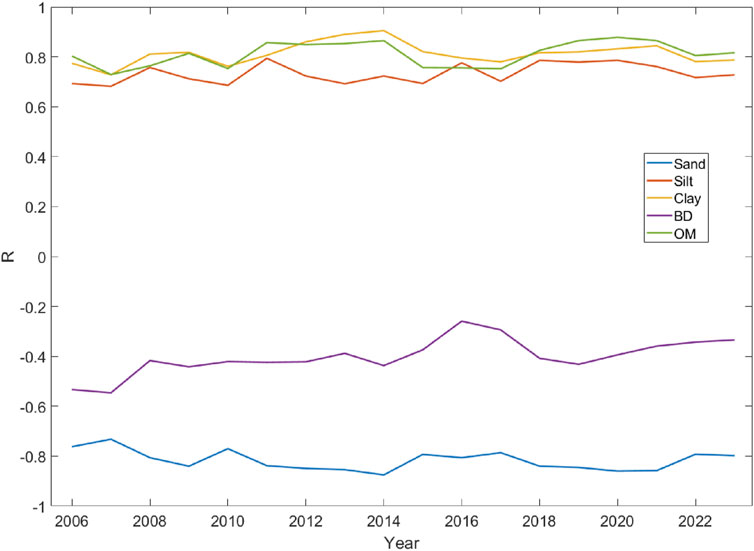
Figure 5. Evolution of the annual Spearman’s correlation coefficient between the mean relative differences (MRD) and soil factors (sand, silt, clay, bulk density (BD), and organic matter (OM)). All the R values are significant (p < 0.01) except for those for BD.
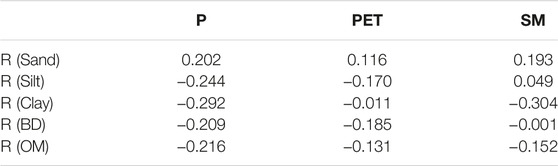
Table 2. Spearman’s correlation coefficient between the R values of the mean relative differences (MRD) of each year and soil factors [texture, bulk density (BD), and organic matter content (OM)], annual mean precipitation (P), potential evapotranspiration (PET) and soil moisture (SM).
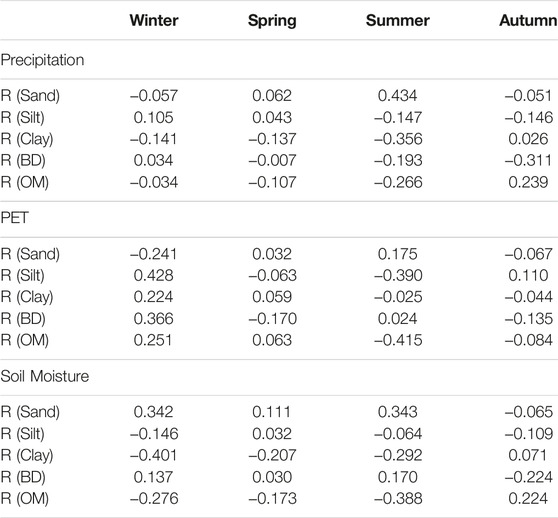
Table 3. Spearman’s correlation coefficient between the R values of the mean relative differences (MRD) of each year and soil factors [texture, bulk density (BD), and organic matter content (OM)], seasonal mean precipitation (P), potential evapotranspiration (PET) and soil moisture (SM).
Similarly, we investigated whether the differences shown in Figure 3 could be related to the hydroclimatic conditions in the study area and their dynamics. For this purpose, the R values of the comparisons between the MRD values of the complete data series and the data series of each year were correlated with the mean values of precipitation, PET, and soil moisture, both at the annual and seasonal scales (Table 4). The results showed that, only in the case of the annual precipitation was a statistically significant R value obtained. Neither the mean soil moisture nor PET was related to the annual variations in the temporal stability pattern.
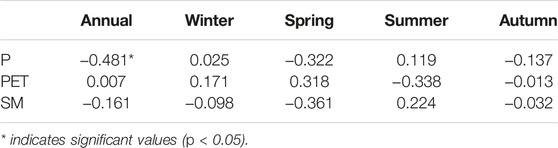
Table 4. Spearman’s correlation coefficient between the R values (mean relative difference of each year vs. entire series) and hydroclimatic factors (precipitation, P; potential evapotranspiration, PET; and soil moisture, SM) at the annual and seasonal scales.
Although there is a certain degree of scatter, this relationship with the average annual precipitation is clearly shown in Figure 6. This indicated that, in general, as the years become drier, the annual pattern becomes more similar to the average pattern. Conversely, as the annual precipitation increases, the two patterns become more distinct. This finding is consistent with the spatial variability pattern observed when comparing the mean soil moisture content with the variance in the study area. The comparative analysis was conducted on a daily scale in the REMEDHUS network for the entire 18-year data series (Figure 7). The relationship obtained is a direct one, and the spatial variability increases with increasing soil water content. This type of relationship is well known and occurs in soils in water-limited areas (Famiglietti et al., 1998; Gómez-Plaza et al., 2000). This pattern differs from that observed in humid environments and is bimodal in nature, with a direct relationship occurring when the soil water content is low and an inverse relationship occurring above a certain threshold, indicating the presence of high soil moisture contents (Brocca et al., 2012; Scaife et al., 2021).
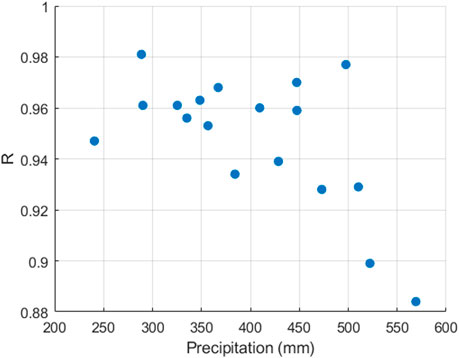
Figure 6. Relationship between the annual precipitation and Spearman’s correlation coefficient between the mean relative differences (MRD) obtained for the entire period (2006–2023) and that obtained for each year in the series.
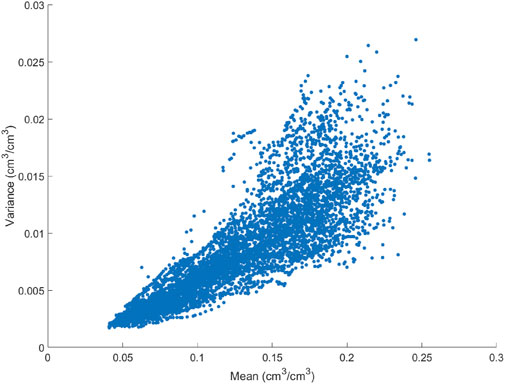
Figure 7. Relationship between the daily mean soil moisture and variance in the REMEDHUS area (2006–2023).
However, in view of the above relationship, Figure 6 shows that the driest year in the series is not the year with the highest R value. This year is 2017, an exceptional year in which only 240 mm of precipitation was recorded. Moreover, in the same year, although winter (−35%), spring (−59%) and autumn (−67%) clearly exhibited below-average rainfall levels, as expected, in summer, rainfall was exceptionally high, 53% higher than the summer season average. This led to the study of the variability in the soil water content in summer as a function of the magnitude of precipitation. To this end, the relationship between soil moisture and variance was analyzed, distinguishing between dry and rainy summers.
The analysis results showed that in cases with below-average precipitation (Figure 8A), the variability pattern coincided with the standard of the area, i.e., the higher the soil water content was, the greater the variability. However, when summer precipitation was higher than the average value (as was the case in 2017), the behavior was anomalous (Figure 8B), without a defined pattern. The high spatial variability in summer rainfall episodes, combined with the very low soil water content during this season, led to a notable change in the spatial distribution pattern of soil moisture. When the spatial variability in rainfall in the study area was analyzed, the distinct characteristics of summer could be highlighted. Figure 9 shows the daily average coefficient of variation (CV) of the daily rainfall for each season obtained from the four weather stations located in the REMEDHUS area. The CV values were high all year, as is characteristic of Mediterranean regions (Goldreich, 1995; Markonis et al., 2017), but summer stood out, with an average value above 100%. These results conform with previous works also reporting higher spatial variability in rainfall in summer under Mediterranean conditions (Cortesi et al., 2014). Even though the number of years used in this study was not large enough to obtain definitive conclusions, all of the findings indicated that rainfall in summer, when water conditions are poor, has relevant importance in altering temporal stability patterns.
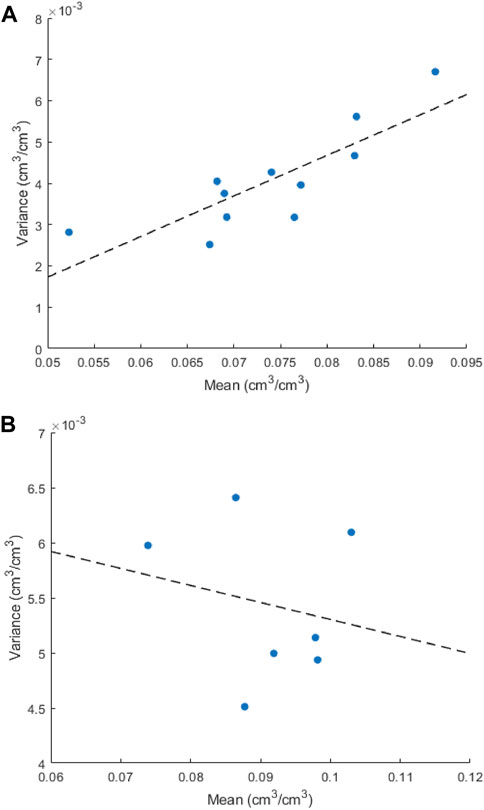
Figure 8. Relationship between the average daily mean soil moisture and variance in the summer months (JJA) when precipitation is lower (A) or above the average (B).
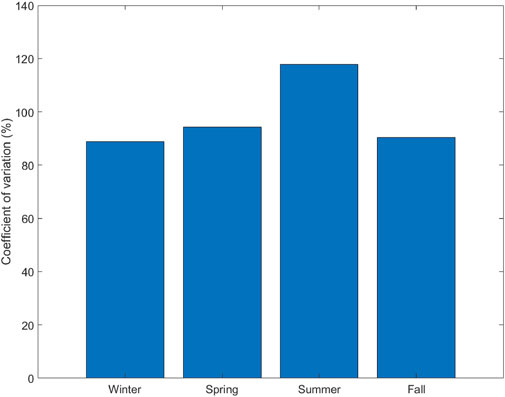
Figure 9. Seasonal distribution of the coefficient of variation of the daily precipitation at the four weather stations located in the REMEDHUS area.
Summer rainfall in arid, semiarid and dry regions, such as the Mediterranean, is usually characterized by irregularities in both time and space, with highly localized episodes of high intensity often predominating (Llasat, 2009). Recent studies have shown the occurrence of a positive trend in the generation of convective episodes in regions with a Mediterranean climate (Llasat et al., 2021), which are the most frequent in summer, and in the intensity and spatiotemporal variability in precipitation (Serrano-Notivoli et al., 2018). Additionally, in some areas, specifically in Spain, the summer duration has increased in recent decades, and it is expected to increase in the near future (Lorenzo and Álvarez, 2022). All of these factors could contribute to an increase in the variability in the spatial distribution patterns of soil moisture.
Conclusion
In this study carried out in REMEDHUS, annual variations in the temporal stability pattern were observed when compared to the pattern of the complete data series. In fact, in more than half of the years studied, the representative station differed from that obtained from the average pattern. Additionally, a clear pattern of the standard deviation of the mean relative differences was observed in the summer months when the sampling points were less temporally stable.
Although the detected variations were not extremely notable, the validity of the assumption of a permanent nature attributed to the pattern of the temporal stability of soil moisture should be assessed.
The influence of soil characteristics on the maintenance of temporal stability was also analyzed. The results showed that this influence was maintained over time with slight variations. It was also observed that the hydroclimatic conditions did not significantly affect this relationship. However, the maximum correlation was obtained with the amount of summer rainfall and the sand fraction, which could indicate an accentuating effect of the decisive role played by the soil texture.
The analysis of the direct influence of hydroclimatic conditions on the variations in the soil moisture stability pattern revealed a clear relationship only in the case of the annual precipitation. The rainier the year is, the more pronounced the difference. Moreover, summer rainfall conditions, specifically the type and amount of precipitation, could influence the change in the temporal stability pattern of soil moisture. In the case study, under water-limited conditions, all the information indicated the determining role of precipitation and, specifically, the behavior in summer. However, this study covered a limited number of years of observations. For this reason, it would be advisable to perform similar analyses with longer-duration soil moisture data series and under different hydroclimatic conditions.
These types of investigations have attracted much interest because their findings have clear implications for research in fields such as hydrological modeling, remote sensing, or precision agriculture. These investigations can be very useful for addressing relevant research issues, such as calibration and validation methodologies, coupling between land and atmosphere systems, memory of hydrological systems and many more.
Data Availability Statement
Publicly available datasets were analyzed in this study. This data can be found here: https://ismn.earth/en/.
Author Contributions
JM-F conceived the general idea and the conceptualization of the study. AG-Z and PB-V performed the statistical tests and developed figures and tables. JM-F wrote the first versions of the manuscript. All authors reviewed and contributed to the writing process. All authors contributed to the article and approved the submitted version.
Funding
The author(s) declare that financial support was received for the research, authorship, and/or publication of this article. This study was funded by the Spanish Ministry of Science, Innovation and Universities MCIN/AEI/10.13039/501100011033/(project PID 2020-114623RB-C33), and the Castilla y León Government (projects SA112P20 and CLU-2018-04), and the European Regional Development Fund (ERDF). PB-V’s research has been funded by a predoctoral grant (FPU20/00592) from the Spanish Ministry of Science, Innovation and Universities.
Conflict of Interest
The authors declare that the research was conducted in the absence of any commercial or financial relationships that could be construed as a potential conflict of interest.
References
Allen, R. G., Pereira, L. S., Raes, D., and Smith, M. (1998). Crop Evapotranspiration-Guidelines for Computing Crop Water Requirements-FAO. Irrigation and Drainage Paper 56, 300. Rome: FAO. (9), D05109.
Basu, R., Daly, E., Brown, C., Shnel, A., and Tuohy, P. (2024). Temporal Stability of Grassland Soil Moisture Utilising Sentinel-2 Satellites and Sparse Ground-Based Sensor Networks. Remote Sens. 16, 220. doi:10.3390/rs16020220
Brocca, L., Tullo, T., Melone, F., Moramarco, T., and Morbidelli, R. (2012). Catchment Scale Soil Moisture Spatial–Temporal Variability. J. Hydrol. 422-423, 63–75. doi:10.1016/j.jhydrol.2011.12.039
Burns, T. T., Berg, A. A., Cockburn, J., and Tetlock, E. (2016). Regional Scale Spatial and Temporal Variability of Soil Moisture in a Prairie Region. Hydrol. Process 30, 3639–3649. doi:10.1002/hyp.10954
Castellini, M., Stellacci, A. M., Tomaiuolo, M., and Barca, E. (2019). Spatial Variability of Soil Physical and Hydraulic Properties in a Durum Wheat Field: An Assessment by the BEST-Procedure. Water 11, 1434. doi:10.3390/w11071434
Ceballos, A., Martínez-Fernández, J., Santos, F., and Alonso, P. (2002). Soil-Water Behaviour of Sandy Soils Under Semi-Arid Conditions in the Duero Basin (Spain). J. Arid. Environ. 51, 501–519. doi:10.1016/s0140-1963(02)90973-0
Coopersmith, E. J., Cosh, M. H., Starks, P. J., Bosch, D. D., Holifield Collins, C., Seyfried, M., et al. (2021). Understanding Temporal Stability: A Long-Term Analysis of USDA ARS Watersheds. Int. J. Digit. Earth 14, 1243–1254. doi:10.1080/17538947.2021.1943550
Cortesi, N., González-Hidalgo, J. C., Brunetti, M., and de Luis, M. (2014). Spatial Variability of Precipitation in Spain. Reg. Environ. Change 14, 1743–1749. doi:10.1007/s10113-012-0402-6
Cosh, M. H., Jackson, T. J., Bindlish, R., and Prueger, J. H. (2004). Watershed Scale Temporal and Spatial Stability of Soil Moisture and its Role in Validating Satellite Estimates. Remote Sens. Environ. 92, 427–435. doi:10.1016/j.rse.2004.02.016
Dari, J., Morbidelli, R., Saltalippi, C., Massari, C., and Brocca, L. (2019). Spatial-Temporal Variability of Soil Moisture: Addressing the Monitoring at the Catchment Scale. J. Hydrol. 570, 436–444. doi:10.1016/j.jhydrol.2019.01.014
Dorigo, W., Himmelbauer, I., Aberer, D., Schremmer, L., Petrakovic, I., Zappa, L., et al. (2021). The International Soil Moisture Network: Serving Earth System Science for Over a Decade. Hydrol. Earth Syst. Sci. 25, 5749–5804. doi:10.5194/hess-25-5749-2021
Entin, J. K., Robock, A., Vinnikov, K. Y., Hollinger, S. E., Liu, S., and Namkhai, A. (2000). Temporal and Spatial Scales of Observed Soil Moisture Variations in the Extratropics. J. Geophys. Res. 105 (D9), 11865–11877. doi:10.1029/2000jd900051
Famiglietti, J. S., Rudnicki, J. W., and Rodell, M. (1998). Variability in Surface Moisture Content Along a Hillslope Transect: Rattlesnake Hill, Texas. J. Hydrol. 210, 259–281. doi:10.1016/s0022-1694(98)00187-5
Gao, X., Zhao, X., Cheng Si, B., Brocca, L., Hu, W., and Wu, P. (2015). Catchment-Scale Variability of Absolute Versus Temporal Anomaly Soil Moisture: Time-Invariant Part Not Always Plays the Leading Role. J. Hydrol. 529, 1669–1678. doi:10.1016/j.jhydrol.2015.08.020
Gaona, J., Benito-Verdugo, P., Martínez-Fernández, J., González-Zamora, A., Almendra-Martín, L., and Herrero-Jiménez, C. M. (2023). Predictive Value of Soil Moisture and Concurrent Variables in the Multivariate Modelling of Cereal Yields in Water-Limited Environments. Agric. Water Manag. 282, 108280. doi:10.1016/j.agwat.2023.108280
Goldreich, Y. (1995). Temporal Variations of Rainfall in Israel. Clim. Res. 5, 167–179. doi:10.3354/cr005167
Gómez-Plaza, A., Álvarez-Rogel, J., Albaladejo, J., and Castillo, V. M. (2000). Spatial Patterns and Temporal Stability of Soil Moisture Across a Range of Scales in a Semi-Arid Environment. Hydrol. Process 14, 1261–1277. doi:10.1002/(sici)1099-1085(200005)14:7<1261::aid-hyp40>3.3.co;2-4
González-Zamora, Á., Sánchez, N., and Martínez-Fernández, J. (2016). Validation of Aquarius Soil Moisture Products Over the Northwest of Spain: A Comparison With SMOS. IEEE J. Sel. Top. Appl. Earth Obs. Remote Sens. 9, 2763–2769. doi:10.1109/jstars.2016.2517401
González-Zamora, Á., Sánchez, N., Martínez-Fernández, J., Gumuzzio, A., Piles, M., and Olmedo, E. (2015). Long-Term SMOS Soil Moisture Products: A Comprehensive Evaluation Across Scales and Methods in the Duero Basin (Spain). Phys. Chem. Earth 83-84, 123–136. doi:10.1016/j.pce.2015.05.009
González-Zamora, Á., Sánchez, N., Pablos, M., and Martínez-Fernández, J. (2019). CCI Soil Moisture Assessment With SMOS Soil Moisture and in Situ Data Under Different Environmental Conditions and Spatial Scales in Spain. Remote Sens. Environ. 225, 469–482. doi:10.1016/j.rse.2018.02.010
Guber, A. K., Gish, T. J., Pachepsky, Y. A., van Genuchten, M. T., Daughtry, C. S. T., Nicholson, T. J., et al. (2008). Temporal Stability of Estimated Soil Water Flux Patterns Across Agricultural Fields. Int. Agrophysics 22, 209–214.
Gubler, A., Wächter, D., Schwab, P., Müller, M., and Keller, A. (2019). Twenty-Five Years of Observations of Soil Organic Carbon in Swiss Croplands Showing Stability Overall but With Some Divergent Trends. Environ. Monit. Assess. 191, 277. doi:10.1007/s10661-019-7435-y
Gumuzzio, A., Brocca, L., Sánchez, N., González-Zamora, Á., and Martínez-Fernández, J. (2016). Comparison of SMOS, Modelled and in Situ Long-Term Soil Moisture Series in the Northwest of Spain. Hydrol. Sci. J. 61, 2610–2625. doi:10.1080/02626667.2016.1151981
Hao, X., Qiu, Y., Fan, Y., Li, T., Leng, D., Li, S., et al. (2020). Applicability of Temporal Stability Analysis in Predicting Field Mean of Soil Moisture in Multiple Soil Depths and Different Seasons in an Irrigated Vineyard. J. Hydrol. 588, 125059. doi:10.1016/j.jhydrol.2020.125059
He, Z.-B., Zhao, M. M., Zhu, X., Du, J., Chen, L. F., Lin, P. F., et al. (2019). Temporal Stability of Soil Water Storage in Multiple Soil Layers in High-Elevation Forests. J. Hydrol. 569, 532–545. doi:10.1016/j.jhydrol.2018.12.024
Jones, A. R., and Brunsell, N. A. (2009). Energy Balance Partitioning and Net Radiation Controls on Soil Moisture-Precipitation Feedbacks. Earth Interact. 13, 1–25. doi:10.1175/2009ei270.1
Kachanoski, R. G., and de Jong, E. (1988). Scale Dependence and the Temporal Persistence of Spatial Patterns of Soil Water Storage. Water Resour. Res. 24, 85–91. doi:10.1029/wr024i001p00085
Kim, Y., Park, H., Kimball, J. S., Colliander, A., and McCabe, M. F. (2023). Global Estimates of Daily Evapotranspiration Using SMAP Surface and Root-Zone Soil Moisture. Remote Sens. Environ. 298, 113803. doi:10.1016/j.rse.2023.113803
Lal, R., Bouma, J., Brevik, E., Dawson, L., Field, D. J., Glaser, B., et al. (2021). Soils and Sustainable Development Goals of the United Nations: An International Union of Soil Sciences Perspective. Geoderma Reg. 25, e00398. doi:10.1016/j.geodrs.2021.e00398
Llasat, M. C. (2009). “High Magnitude Storms and Floods,” in The Physical Geography of the Mediterranean. Editor J. C. Woodward (Oxford: Oxford University Press), 513–540.
Llasat, M. C., del Moral, A., Cortés, M., and Rigo, T. (2021). Convective Precipitation Trends in the Spanish Mediterranean Region. Atmos. Res. 257, 105581. doi:10.1016/j.atmosres.2021.105581
Loew, A., and Schlenz, F. (2011). A Dynamic Approach for Evaluating Coarse Scale Satellite Soil Moisture Products. Hydrol. Earth Syst. Sci. 15, 75–90. doi:10.5194/hess-15-75-2011
Lorenzo, M. M., and Álvarez, I. (2022). Future Changes of Hot Extremes in Spain: Towards Warmer Conditions. Nat. Hazards 113, 383–402. doi:10.1007/s11069-022-05306-x
Markonis, Y., Batelis, S. C., Dimakos, Y., Moschou, E., and Koutsoyiannis, D. (2017). Temporal and Spatial Variability of Rainfall Over Greece. Theor. Appl. Climatol. 130, 217–232. doi:10.1007/s00704-016-1878-7
Martínez-Fernández, J., and Ceballos, A. (2003). Temporal Stability of Soil Moisture in a Large-Field Experiment in Spain. Soil Sci. Soc. Am. J. 67, 1647–1656. doi:10.2136/sssaj2003.1647
Martinez-Fernandez, J., and Ceballos, A. (2005). Mean Soil Moisture Estimation Using Temporal Stability Analysis. J. Hydrol. 312, 28–38. doi:10.1016/j.jhydrol.2005.02.007
Martínez-Fernández, J., González-Zamora, Á., and Almendra-Martín, L. (2021). Soil Moisture Memory and Soil Properties: An Analysis With the Stored Precipitation Fraction. J. Hydrol. 593, 125622. doi:10.1016/j.jhydrol.2020.125622
Martínez-Fernández, J., González-Zamora, A., Sánchez, N., Gumuzzio, A., and Herrero-Jiménez, C. M. (2016). Satellite Soil Moisture for Agricultural Drought Monitoring: Assessment of the SMOS Derived Soil Water Deficit Index. Remote Sens. Environ. 177, 277–286. doi:10.1016/j.rse.2016.02.064
Martínez-Fernández, J., Molina-Navarro, E., González-Zamora, A., Sánchez-Gómez, A., and Almendra-Martín, L. (2023). SWAT Soil Moisture Assessment Under Mediterranean Conditions: An Intercomparison Analysis in the Henares Basin (Spain). J. Hydrol-Reg Stud. 48, 101460. doi:10.1016/j.ejrh.2023.101460
Moragoda, N., Kumar, M., and Cohen, S. (2022). Representing the Role of Soil Moisture on Erosion Resistance in Sediment Models: Challenges and Opportunities. Earth-Science Rev. 229, 104032. doi:10.1016/j.earscirev.2022.104032
Nielsen, D. R., Biggar, J. W., and Erh, K. T. (1973). Spatial Variability of Field-Measured Soil-Water Properties. Hilgardia 42 (7), 215–260.
Owe, M., Jones, E. B., and Schmugge, T. J. (1982). Soil Moisture Variation Patterns Observed in Hand County, South Dakota. Water Resour. Bull. 18, 949–954. doi:10.1111/j.1752-1688.1982.tb00100.x
Penna, D., Brocca, L., Borga, M., and Dalla Fontana, G. (2013). Soil Moisture Temporal Stability at Different Depths on Two Alpine Hillslopes During Wet and Dry Periods. J. Hydrol. 477, 55–71. doi:10.1016/j.jhydrol.2012.10.052
Salvati, L., Moretti, V., Zitti, M., and Ferrara, C. (2015). Towards Soil Water Scarcity? An Exploratory Time-Series Analysis of the Aridity Index in Castelporziano Forest, Rome. Rend. Fis. Acc. Lincei 26, 289–295. doi:10.1007/s12210-015-0409-2
Sánchez, N., Martínez-Fernández, J., Calera, A., Torres, E. A., and Pérez-Gutiérrez, C. (2010). Combining Remote Sensing and in Situ Soil Moisture Data for the Application and Validation of a Distributed Water Balance Model (HIDROMORE). Agr. Water Manage 98, 69–78. doi:10.1016/j.agwat.2010.07.014
Sánchez, N., Martínez-Fernández, J., Scaini, A., and Pérez-Gutiérrez, C. (2012). Validation of the SMOS L2 Soil Moisture Data in the REMEDHUS Network (Spain). IEEE Trans. Geosci. Remote Sens. 50, 1602–1611. doi:10.1109/tgrs.2012.2186971
Sánchez-Ruiz, S., Piles, M., Sánchez, N., Martínez-Fernández, J., Vall-llossera, M., and Camps, A. (2014). Combining SMOS With Visible and Near/Shortwave/Thermal Infrared Satellite Data for High Resolution Soil Moisture Estimates. J. Hydrol. 516, 273–283. doi:10.1016/j.jhydrol.2013.12.047
Scaife, C. I., Duncan, J. M., Lin, L., Tague, C., Bell, C. D., and Band, L. (2021). Are Spatial Patterns of Soil Moisture at Plot Scales Generalizable Across Catchments, Climates, and Other Characteristics? A Synthesis of Synoptic Soil Moisture Across the Mid-Atlantic. Hydrol. Process 35, e14313. doi:10.1002/hyp.14313
Sepulcre-Canto, G., Horion, SMAF, Singleton, A., Carrao, H., and Vogt, J. (2012). Development of a Combined Drought Indicator to Detect Agricultural Drought. Nat. Hazards Earth Syst. Sci. 12, 35193531. doi:10.5194/nhess-12-3519-2012
Serrano-Notivoli, R., Martín-Vide, J., Saz, M. A., Longares, L. A., Beguería, S., Sarricolea, P., et al. (2018). Spatio-Temporal Variability of Daily Precipitation Concentration in Spain Based on a High-Resolution Gridded Data Set. Int. J. Climatol. 38, e518–e530. doi:10.1002/joc.5387
Singh, D., Mishra, A. K., Patra, S., Mariappan, S., Singh, N., and Kar, S. K. (2013). Spatial Variability of Soil Hydraulic and Physical Properties in Erosive Sloping Agricultural Fields. Water Sci. Eng. 16, 57–66. doi:10.1016/j.wse.2022.10.001
Su, F., Wu, J., Wang, D., Zhao, H., Wang, Y., and He, X. (2022). Moisture Movement, Soil Salt Migration, and Nitrogen Transformation Under Different Irrigation Conditions: Field Experimental Research. Chemosphere 300, 134569. doi:10.1016/j.chemosphere.2022.134569
Vachaud, G., Passerat de Silans, A., Balabanis, P., and Vauclin, M. (1985). Temporal Stability of Spatially Measured Soil Water Probability Density Function. Soil Sci. Soc. Am. J. 49, 822–828. doi:10.2136/sssaj1985.03615995004900040006x
Vanderlinden, K., Vereecken, H., Hardelauf, H., Herbst, M., Martínez, G., Cosh, M. H., et al. (2012). Temporal Stability of Soil Water Contents: A Review of Data and Analyses. Vadose Zone J. 11. vzj2011.0178. doi:10.2136/vzj2011.0178
Wagner, W., Pathe, C., Doubkova, M., Sabel, D., Bartsch, A., Hasenauer, S., et al. (2008). Temporal Stability of Soil Moisture and Radar Backscatter Observed by the Advanced Synthetic Aperture Radar (ASAR). Sensors 8, 1174–1197. doi:10.3390/s80201174
Wang, T., Liu, Q., Franz, T. E., Li, R., Lang, Y., and Fiebrich, C. A. (2017). Spatial Patterns of Soil Moisture From Two Regional Monitoring Networks in the United States. J. Hydrol. 552, 578–585. doi:10.1016/j.jhydrol.2017.07.035
Keywords: soil moisture, Mediterranean conditions, temporal stability, mean relative differences, soil properties, climatic factors
Citation: González-Zamora Á, Benito-Verdugo P and Martínez-Fernández J (2024) On the Variability in the Temporal Stability Pattern of Soil Moisture Under Mediterranean Conditions. Span. J. Soil Sci. 14:12839. doi: 10.3389/sjss.2024.12839
Received: 12 February 2024; Accepted: 29 May 2024;
Published: 10 June 2024.
Edited by:
Daniel Dearmond, National Institute of Amazonian Research (INPA), BrazilCopyright © 2024 González-Zamora, Benito-Verdugo and Martínez-Fernández. This is an open-access article distributed under the terms of the Creative Commons Attribution License (CC BY). The use, distribution or reproduction in other forums is permitted, provided the original author(s) and the copyright owner(s) are credited and that the original publication in this journal is cited, in accordance with accepted academic practice. No use, distribution or reproduction is permitted which does not comply with these terms.
*Correspondence: Ángel González-Zamora, YWdsZXp6YW1vcmFAdXNhbC5lcw==