- Laboratory of Intracellular Ion Channels, Nencki Institute of Experimental Biology, Polish Academy of Sciences, Warsaw, Poland
Mitochondrial investigations have extended beyond their traditional functions, covering areas such as ATP synthesis and metabolism. Mitochondria are now implicated in new functional areas such as cytoprotection, cellular senescence, tumor function and inflammation. The basis of these new areas still relies on fundamental biochemical/biophysical mitochondrial functions such as synthesis of reactive oxygen species, mitochondrial membrane potential, and the integrity of the inner mitochondrial membrane i.e., the passage of various molecules through the mitochondrial membranes. In this view transport of potassium cations, known as the potassium cycle, plays an important role. It is believed that K+ influx is mediated by various potassium channels present in the inner mitochondrial membrane. In this article, we present an overview of the key findings and characteristics of mitochondrial potassium channels derived from research of many groups conducted over the past 33 years. We propose a list of six fundamental observations and most important ideas dealing with mitochondrial potassium channels. We also discuss the contemporary challenges and future prospects associated with research on mitochondrial potassium channels.
Introduction
When investigating the fundamentals of mitochondrial function within cells, we can identify several simple cations that form the basis of many processes (Szabo and Zoratti, 2014). It is well known that the proton gradient serves as the driving force for ATP synthesis in mitochondria. The Ca2+ cations entering the mitochondria not only buffer the cytosolic pool of these ions but can also contribute to some physiological situations such as the mitochondrial mega-channel activation (Carraro and Bernardi, 2023; Zoratti et al., 2024). The effects of Mg2+ on mitochondrial functions such as energy metabolism, mitochondrial Ca2+ handling, and apoptosis are well established (Liu and Dudley, 2020). Mitochondrial Na+ have been discovered as a new second messenger regulating inner mitochondrial membrane (IMM) fluidity and reactive oxygen species (ROS) generation by respiratory chain complex III (Hernansanz-Agustín and Enríquez, 2022). In this study, we will focus on the properties and the role of K+ transport, via potassium channels (mitoK channels) present in IMM (Szewczyk, 1996; Kicinska et al., 2000; Debska et al., 2001; O’Rourke, 2007; Singh et al., 2012; Szabo and Szewczyk, 2023) (Figure 1).
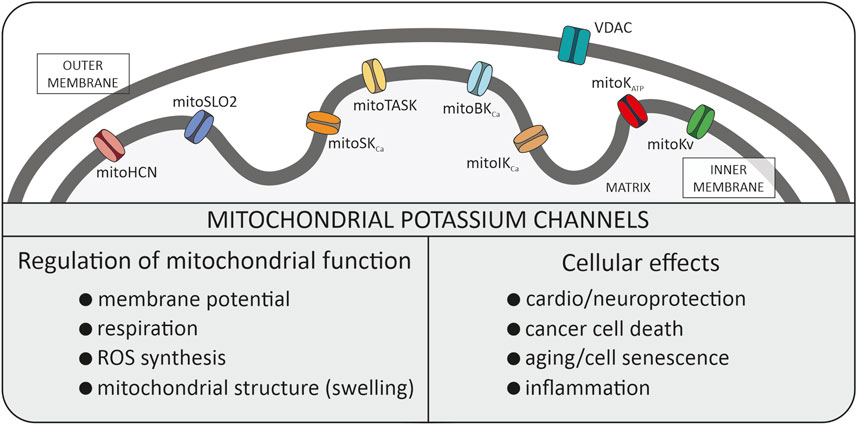
Figure 1. Potassium channels identified in the inner mitochondrial membrane. All these types of channels were described in the manuscript. Additionally, the biophysical role in mitochondria and physiological role within the cell is summarized. VDAC, voltage dependent anion channel (porin).
In general, proper mitochondrial function is based on the integrity of mitochondrial membranes. Peter Mitchell in his Nobel Lecture delivered in 1978 underlined the importance of the low permeability of the IMM to ions (Mitchell, 1985). Consequently, the discovery of multiple potassium channels in the IMM was for many years considered to be an experimental artifact. Nowadays, mitoK channels present in the IMM are recognized as crucial players for regulating some mitochondrial function (Kravenska et al., 2021; Szabo and Szewczyk, 2023). The mitoK channels have attracted attention for many years, especially in the context of the regulation of life/death processes in the various cell types (Garlid, 2000). For example, the activation of mitoK channels may induce cytoprotective phenomena in cardiac tissue and in neurons (O’Rourke, 2007). On the contrary, inhibition of mitoK channels may cause cell death (Checchetto et al., 2021).
In this paper, we will present what we consider to be the most significant discoveries/ideas in the field of mitoK channels over the past 33 years. These subjective, proposed by author, list of six the most important observations are as follows: 1). Discovery of mitoK channels in various tissues and identification of their molecular identity; 2). Cytoprotection (cardioprotection, neuroprotection) induced by mitoK channels activation; 3). Cancer cell death by mitoK channels inhibition; 4). Role of mitoK channels in aging/senescence/life span; 5). Interactions of mitoK channels with respiratory chain; 6). Druggability of the mitoK channels.
Mitochondrial potassium channel discovery
In 1991, an ion channel selective for K+ was discovered in the IMM of rat liver mitochondria (Inoue et al., 1991), confirming previous findings on channels in mitochondria by Catia Sorgato (Sorgato et al., 1987). However, what significantly altered the interpretation of this experimental data was the revelation that the channel exhibited not only K+ selectivity but also susceptibility to inhibition by ATP and the antidiabetic sulfonylurea, glibenclamide (Inoue et al., 1991). This discovery situated mitoK channels within a similar family to ATP-regulated potassium channels found in the plasma membrane of pancreatic beta-cells, cardiomyocytes, neurons, and others (Szewczyk, 1996; O’Rourke, 2007). Undoubtedly, this observation served as a pivotal starting point for numerous experiments identifying ATP-regulated mitochondrial potassium (mitoKATP) channels across various tissues, notably cardiomyocytes (Szewczyk et al., 2009; Szabo and Szewczyk, 2023). Following several years of intensive investigation across multiple laboratories into the functional role of these channels, it was demonstrated that the activation of mitoKATP channels (by potassium channel openers) induces a cardioprotective phenomenon (Liu et al., 1999; Garlid, 2000; Szteyn and Singh, 2020; Lukowski et al., 2022). Subsequently, similar findings in neural tissue suggested the involvement of these channels in neuroprotection (Busija et al., 2004; Bednarczyk, 2009). In summary, the association of mitoKATP channels with cytoprotection marked a significant milestone in the rapid development of the mitochondrial potassium channel field. Moreover, other mitoK channels (such as large conductance calcium-activated potassium—mitoBKCa channels) were later implicated in cytoprotection across various cell types (Xu et al., 2002). Despite a plethora of observations, however, the biochemical mechanisms underlying these events remain unclear. It is probable that the indirect modulation of ROS generation by mitoK channels (via depolarization of mitochondria) contributes to this phenomenon.
In recent years, researchers have demonstrated that the family of mitoK channels constitutes one of the most numerous classes of mitochondrial channel proteins. They are also present in plants and in simple organisms (Koszela-Piotrowska et al., 2009; Matkovic et al., 2011; Laskowski et al., 2015). It includes not only mitoKATP and mitoBKCa channels but also intermediate conductance (mitoIKCa), and small conductance (mitoSKCa), voltage-regulated potassium (mitoKv1.3, mitoKv7.4) channels, mitochondrial hyperpolarization-activated cyclic nucleotide-gated (mitoHCN) channels, mitochondrial sodium-activated potassium (mitoSlo2) channel and two-pore domain potassium (mitoTASK-3) channel (Szabo and Szewczyk, 2023). The activity of potassium channels are regulated by various stimuli, such as pH, Ca2+ and ROS (Szabo and Szewczyk, 2023). The mitoK channels have been identified in many tissues but at the same time their number of molecules in mitochondrial membranes is relatively small compared to other mitochondrial transport proteins. Probably low density of mitoK channels and channel run down phenomenon were reasons for questioning in the 90’s the presence of these channels at all.
Another issue regarding mitoK channels is the following: why is such a simple process, like K+ influx into a matrix, facilitated by a wide variety of potassium channels? For example, in cardiac mitochondria, six mitoK channels have been identified: mitoKATP, mitoBKCa, mitoSKCa, mitoSlo2, mitoHCN channels and mitoKv7.4 channels (Szabo and Szewczyk, 2023). What is the physiological benefit of using many different ligands and factors to regulate these channels? Probably, potassium channels present in cardiomyocyte mitochondria are activated under specific physiological circumstances (Kulawiak et al., 2021). An early event during cardiac ischemia is ATP depletion. This is followed by mitochondrial membrane depolarization. Moreover, because of ATP depletion, ion pumps cannot function, leading to an increase in the cellular Ca2+ concentration. The rise in Ca2+ during ischemia and reperfusion leads to an overload of mitochondrial Ca2+, during reperfusion when oxygen is reintroduced. The decrease in intracellular pH during severe ischemia promotes the imbalance of other cations and leading to cellular Na+ overload (Kulawiak et al., 2021). These complex changes may lead to channel activation/inhibition possibly explains why there are few potassium channels in cardiac mitochondria. Most likely, the timing of ATP, pH, Ca2+, and Na+ concentration changes is critical to control K+ flux in mitochondria stabilizing structure of mitochondria.
Molecular identity of mitoK channels for many years was a mystery. Lack of molecular mitoK identity was an argument questioning the presence of potassium channels in mitochondria. Let’s summarize this long way of channel molecular identity recognition. Today we believe that mitoBKCa channel is one of the splice variants of KCNMA1 (Slo1) gene (Singh et al., 2013; Galecka et al., 2021). Properties of mitoBKCa suggest that the pore-forming subunit is encoded by the same gene coding for plasma membrane BKCa. Several studies suggested that the VEDEC BKCa isoform is located in the IMM. With the mitoKATP channel there is a more complex situation. It can not be excluded that K+ influx is catalyzed by 2-3 various proteins in various tissues. Recently, it was shown that the pore-forming subunit of the mitoKATP channel is a product of the CCDC51 gene (Paggio et al., 2019). The mitoKATP is inhibited by the antidiabetic sulfonylurea glibenclamide. Therefore, it was speculated that the glibenclamide receptor (product of ABC8/MITOSUR gene) is an integral part of the mitoK channel. Indeed the mitoKATP channel formed by these two proteins has the established pharmacological properties of the mitoKATP channel (Paggio et al., 2019). Previous studies showed that also the ROMK2 potassium channel isoform of the renal outer medullary potassium channel could be the component of the mitoKATP channel (Bednarczyk et al., 2018; Laskowski et al., 2019). Detailed discussion on mitochondrial potassium channel molecular identity was recently reviewed (Szabo and Szewczyk, 2023).
The presence of various auxiliary β subunits in mitoBKCa channels and sulfonylurea receptors in the mitoKATP channel causes that, despite undoubted progress in the identification of channel proteins, the problem of their detailed identification is still a challenge for the future (Piwonska et al., 2008).
From cytoprotection to cell death
The mitoK channels have been described as an important player in cellular pro-life and death signaling. The activation of mitoK channels (by potassium channel openers), such as ATP-regulated or calcium-activated large conductance potassium channels, may have cytoprotective effects in cardiac or neuronal tissue (Liu et al., 1999; Busija et al., 2004). This concept was a strong driving force of studies in many laboratories. Potassium channel opener induced cytoprotection is also induced by endogenous signaling via protein kinases (Frankenreiter et al., 2017).
It has also been shown that inhibition with channel blockers of the mitochondrial Kv1.3 channel may lead to pancreatic cancer cell death (Leanza et al., 2014). But still there is an open question to what extent mitoK channels are promising drug targets in various organs and tissues? Future prospects of the druggability concept of mitoK channels was evaluated recently (Wrzosek et al., 2020).
Searching for new functions of mitochondrial potassium channels
The putative functional roles of these channels involve alterations in mitochondrial matrix volume, mitochondrial respiration, and protonmotive force (membrane potential) (Czyz et al., 1995). Furthermore, the activity of these channels influences the generation of ROS by mitochondria (Kulawiak et al., 2008; Kulawiak et al., 2023). The activity of mitochondrial potassium channels is subject to modulation by various intrinsic signals, including Ca2+ concentration, membrane potential, phosphorylation, and membrane stretching (Szabo and Szewczyk, 2023).
It was demonstrated that BKCa channels are present in Drosophila melanogaster mitochondria, and channel mutants induce structural and functional defects in mitochondria leading to an increase in ROS (Gururaja Rao et al., 2019). It was found that the absence of BKCa channels reduced the lifespan of Drosophila, and overexpression of human BKCa channels in flies extends their life. This suggested a potential role of mitoK channels and ROS in regulating mitochondrial functional integrity, and lifespan (Gururaja Rao et al., 2019). Probably mitoBKCa play a role in cellular senescence induced by oxidative stress (Gluchowska et al., 2023).
Mitochondrial context of potassium channel regulation
The mitochondrial respiratory chain comprises a series of complex organized redox reactions generating a protonmotive force and, consequently, ATP synthesis. Certain redox centers, such as complexes I and III of the mitochondrial respiratory chain are sources of ROS. Mitochondrial generated ROS can influence remotely the activity of mitoK channels. But there are some indications proposing an alternative, a direct mechanism for the regulation of mitoK channels by the respiratory chain.
It is well-known that mitoK channels interact with various mitochondrial proteins, some of which are involved in the respiratory chain. These observations were recently summarized (Lewandowska et al., 2024). For instance, it has been suggested that mitoKATP channels interact with succinate dehydrogenase. In cardiac mitochondria, it was found that the β1 subunit of the mitoBKCa channels interacts with Cytochrome c Oxidase (COX) subunit I. Furthermore, studies have demonstrated that other respiratory chain protein complexes interact with mitoBKCa channels in both cardiac and brain mitochondria. Additionally, mitochondrial tandem pore domain K+ channels TASK-3 interact also with the respiratory chain. A recent report revealed a similar interaction between the mitoKv1.3 channel and respiratory chain complex I (for review see Lewandowska et al., 2024).
We found that the activity of mitoBKCa channels in glioblastoma cells is regulated by substrates and inhibitors of the respiratory chain (Bednarczyk et al., 2013). This study suggested that COX is a key element of this kind of channel regulation (Bednarczyk et al., 2013). Moreover, given that COX is the primary infrared-absorbing protein, it raises questions about the potential light regulation of mitoK channels (Szewczyk and Bednarczyk, 2018).
Further research will be important to clarify the functional consequences of these interactions. Undoubtedly, this form of regulation may prove to be unique for mitoK channels. The exact nature and functional implications of these interactions remain unclear. This kind of direct functional coupling between the energy generating system (respiratory chain) with the energy dissipation system (potassium channels) may lead to an interesting putative regulatory mechanism in mitochondria.
Recently other functional/structural coupling within the mitochondrial potassium channel was observed. It was found that mitochondrial potassium channel ROMK2 may interact with two lipid kinases: acylglycerol kinase (AGK) and diacylglycerol kinase ε (DGKE), which are localized in mitochondria (Krajewska et al., 2024). Additionally, it was found that the products of AGK and DGKE, lysophosphatidic acid (LPA) and phosphatidic acid (PA), stimulated the activity of ROMK2 potassium channels reconstituted in planar lipid bilayers (Krajewska et al., 2024).
The structure/function interplay of mitoK channels alongside other mitochondrial proteins suggests a new dimension in mitoK channels regulation. The exceptionally high membrane potential of the IMM and its potential for ROS generation may characterize significant signaling pathways within cells.
The troublesome pharmacology of mitochondrial potassium channels
In order to influence activity of various mitoK channels, numerous research groups continually explore novel compounds hoping to find molecules with high specificity for mitoK channels (Szewczyk and Marban, 1999; Augustynek et al., 2017; Leanza et al., 2019). The existing literature already reports positive protective effects on ischemia/reperfusion processes through the activation of mitoKATP channels by the potassium channel opener - diazoxide, and the mitoBKCa channels by potassium channel opener NS1619 and its follower NS11021 (Szewczyk et al., 2006). Nevertheless, it is noteworthy that these compounds exhibit limited specificity towards mitoK channels. Application of these substances in the micromolar concentration range unmasks a variety of side effects (Wrzosek et al., 2022). It is important to remember that molecules with some hydrophobicity of positive charge (in physiological pH) will be accumulated by mitochondria. It is due to very high membrane potential (up to—180 mV) on the IMM, with negative polarization of the mitochondrial matrix. For example, a 10 nM drug present in cytosol could accumulate up to 10 µM concentration in a matrix (Kowaltowski and Adbulkader, 2024). At this concentration range the probability of nonspecific interaction with some of ∼1,500 mitochondrial proteins is very high. In contrast, toxins isolated from the venom of various scorpion species such as iberiotoxin specifically (at low concentration) inhibit the activity of mitoBKCa channels (Augustynek et al., 2017). But application of this peptide to block mitoBKCa channels on intact cells is practically impossible.
Developing very selective channel blockers and potassium channel openers targeting mitoK channels is a significant challenge in this field. Recently it was shown that selective targeting of mitoIKCa channel (Bachmann et al., 2022), mitoTASK channel (Bachmann et al., 2021) and mitoKv channel (Severin et al., 2022) is possible.
Discussion
Over the past 33 years since the identification of the first potassium channel in the IMM, research in this field has made significant progress (Kulawiak and Szewczyk, 2022). This pathway started from identification of the mitoK channels that met with skepticism by the bioenergetics community to current research placing these channels in the phenomena of cytoprotection, cellular senescence, and neoplastic cell death. What limits further development of this field?
First, access to good pharmacology is the “dark side” of this field (Szewczyk et al., 2010; Olszewska and Szewczyk, 2013; Leanza et al., 2019). Because mitoK channels are similar to those located in plasma membranes, it is very difficult to identify pharmacological modulators specific only for mitoK channels (Szewczyk and Wojtczak, 2002; Citi et al., 2018). The unique high membrane potential of mitochondria may help to discriminate targeting of some drugs to mitoK channels (Testai et al., 2015; Wrzosek et al., 2020).
The second limiting factor for further progress is the development of new techniques to measure channel activity in situ, that is, within an intact cell. Majority of techniques currently applied in the studies are based on cell fractionation and mitochondria isolation (Walewska et al., 2022). By definition in this process we lose a network of signaling pathways where mitoK channels are potentially involved (Walewska et al., 2018). Probably progress in synthesis of potassium specific fluorescent probes may solve this problem. Unfortunately, there are other potassium transport proteins in mitochondria.
The third challenge for the future involves further identifying the molecular identity of various mitoK channels. This aim will not only expand our understanding of the system but also will start new avenues of research, such as in vitro translation with lipid nanodiscs and the application of various biophysical techniques. Additionally, it will aid in the identification of protein neighborhoods, clarification of the import machinery, and more.
In summary, mitoK channels, considered the “younger siblings” of the potassium channels found in plasma membranes, play a crucial role in some cellular signaling pathways. The mitoK location within mitochondria, which serve as hubs for fundamental metabolic and signaling functions, highlight their significance. The author believes that the future of this field holds exciting prospects.
Author contributions
The author confirms being the sole contributor of this work and has approved it for publication.
Funding
The author(s) declare that financial support was received for the research, authorship, and/or publication of this article. Supported by the Polish National Science Centre (MAESTRO grant No. 2019/34/A/NZ1/00352).
Conflict of interest
The author declares that the research was conducted in the absence of any commercial or financial relationships that could be construed as a potential conflict of interest.
Acknowledgments
This article is dedicated to Prof. Lech Wojtczak (Nencki Institute of Experimental Biology, Warsaw), whose teachings have greatly contributed to my understanding of mitochondria, and to Prof. Michel Lazdunski (Institute of Molecular and Cellular Pharmacology CNRS, Sophia Antipolis), who introduced me to potassium channels.
References
Augustynek, B., Kunz, W. S., and Szewczyk, A. (2017). Guide to the pharmacology of mitochondrial potassium channels. Handb. Exp. Pharmacol. 240, 103–127. doi:10.1007/164_2016_79
Bachmann, M., Rossa, A., Antoniazzi, G., Biasutto, L., Carrer, A., Campagnaro, M., et al. (2021). Synthesis and cellular effects of a mitochondria-targeted inhibitor of the two-pore potassium channel TASK-3. Pharmacol. Res. 164, 105326. doi:10.1016/j.phrs.2020.105326
Bachmann, M., Rossa, A., Varanita, T., Fioretti, B., Biasutto, L., Milenkovic, S., et al. (2022). Pharmacological targeting of the mitochondrial calcium-dependent potassium channel KCa3.1 triggers cell death and reduces tumor growth and metastasis in vivo. Cell death Dis. 13 (12), 1055. doi:10.1038/s41419-022-05463-8
Bednarczyk, P. (2009). Potassium channels in brain mitochondria. Acta Biochim. Pol. 56, 385–392. doi:10.18388/abp.2009_2471
Bednarczyk, P., Kicinska, A., Laskowski, M., Kulawiak, B., Kampa, R., Walewska, A., et al. (2018). Evidence for a mitochondrial ATP-regulated potassium channel in human dermal fibroblasts. Biochim. Biophys. Acta Bioenerg. 1859, 309–318. doi:10.1016/j.bbabio.2018.02.005
Bednarczyk, P., Wieckowski, M. R., Broszkiewicz, M., Skowronek, K., Siemen, D., and Szewczyk, A. (2013). Putative structural and functional coupling of the mitochondrial BKCa channel to the respiratory chain. PLoS One 8 (6), e68125. doi:10.1371/journal.pone.0068125
Busija, D. W., Lacza, Z., Rajapakse, N., Shimizu, K., Kis, B., Bari, F., et al. (2004). Targeting mitochondrial ATP-sensitive potassium channels--a novel approach to neuroprotection. Brain Res. Rev. 46, 282–294. doi:10.1016/j.brainresrev.2004.06.011
Carraro, M., and Bernardi, P. (2023). The mitochondrial permeability transition pore in Ca2+ homeostasis. Cell calcium 111, 102719. doi:10.1016/j.ceca.2023.102719
Checchetto, V., Leanza, L., De Stefani, D., Rizzuto, R., Gulbins, E., and Szabo, I. (2021). Mitochondrial K+ channels and their implications for disease mechanisms. Pharmacol. Ther. 227, 107874. doi:10.1016/j.pharmthera.2021.107874
Citi, V., Calderone, V., Martelli, A., Breschi, M. C., and Testai, L. (2018). Pathophysiological role of mitochondrial potassium channels and their modulation by drugs. Curr. Med. Chem. 25 (23), 2661–2674. doi:10.2174/0929867324666171012115300
Czyz, A., Szewczyk, A., Nalecz, M. J., and Wojtczak, L. (1995). The role of mitochondrial potassium fluxes in controlling the protonmotive force in energized mitochondria. Biochem. Biophysical Res. Commun. 210, 98–104. doi:10.1006/bbrc.1995.1632
Debska, G., Kicińska, A., Skalska, J., and Szewczyk, A. (2001). Intracellular potassium and chloride channels: An update. Acta. Biochim. Pol. 48, 137–144. doi:10.18388/abp.2001_5120
Frankenreiter, S., Bednarczyk, P., Kniess, A., Bork, N. I., Straubinger, J., Koprowski, P., et al. (2017). cGMP-elevating compounds and ischemic conditioning provide cardioprotection against ischemia and reperfusion injury via cardiomyocyte-specific BK channels. Circulation 136, 2337–2355. doi:10.1161/CIRCULATIONAHA.117.028723
Gałecka, S., Kulawiak, B., Bednarczyk, P., Singh, H., and Szewczyk, A. (2021). Single channel properties of mitochondrial large conductance potassium channel formed by BK-VEDEC splice variant. Sci. Rep. 11 (1), 10925. doi:10.1038/s41598-021-90465-3
Garlid, K. D. (2000). Opening mitochondrial K(ATP) in the heart--what happens, and what does not happen. Basic Res. Cardiol. 95, 275–279. doi:10.1007/s003950070046
Głuchowska, A., Kalenik, B., Kulawiak, B., Wrzosek, A., Szewczyk, A., Bednarczyk, P., et al. (2023). Lack of activity of the mitochondrial large-conductance calcium-regulated potassium channels in senescent vascular smooth muscle cells. Mech. Ageing Dev. 215, 111871. doi:10.1016/j.mad.2023.111871
Gururaja Rao, S., Bednarczyk, P., Towheed, A., Shah, K., Karekar, P., Ponnalagu, D., et al. (2019). BKCa (slo) channel regulates mitochondrial function and lifespan in Drosophila melanogaster. Cells 8, 945. doi:10.3390/cells8090945
Hernansanz-Agustín, P., and Enríquez, J. A. (2022). Sodium in mitochondrial redox signaling. Antioxidants Redox Signal. 37, 290–300. doi:10.1089/ars.2021.0262
Inoue, I., Nagase, H., Kishi, K., and Higuti, T. (1991). ATP-sensitive K+ channel in the mitochondrial inner membrane. Nature 352, 244–247. doi:10.1038/352244a0
Kicinska, A., D bska, G., Kunz, W., and Szewczyk, A. (2000). Mitochondrial potassium and chloride channels. Acta Biochim. Pol. 47, 541–551. doi:10.18388/abp.2000_3977
Koszela-Piotrowska, I., Matkovic, K., Szewczyk, A., and Jarmuszkiewicz, W. (2009). A large-conductance calcium-activated potassium channel in potato (Solanum tuberosum) tuber mitochondria. Biochem. J. 424, 307–316. doi:10.1042/BJ20090991
Kowaltowski, A. J., and Abdulkader, F. (2024). How and when to measure mitochondrial inner membrane potentials. Biophysical J. S0006-3495 (24), 00176–180. doi:10.1016/j.bpj.2024.03.011
Krajewska, M., Możajew, M., Filipek, S., and Koprowski, P. (2024). Interaction of ROMK2 channel with lipid kinases DGKE and AGK: potential channel activation by localized anionic lipid synthesis. Biochim. Biophys. Acta Mol. Cell Biol. Lipids 1869, 159443. doi:10.1016/j.bbalip.2023.159443
Kravenska, Y., Checchetto, V., and Szabo, I. (2021). Routes for potassium ions across mitochondrial membranes: a biophysical point of view with special focus on the ATP-sensitive K+ channel. Biomolecules. 11, 1172. doi:10.3390/biom11081172
Kulawiak, B., Bednarczyk, P., and Szewczyk, A. (2021). Multidimensional regulation of cardiac mitochondrial potassium channels. Cells 10, 1554. doi:10.3390/cells10061554
Kulawiak, B., Kudin, A. P., Szewczyk, A., and Kunz, W. S. (2008). BK channel openers inhibit ROS production of isolated rat brain mitochondria. Exp. Neurol. 212, 543–547. doi:10.1016/j.expneurol.2008.05.004
Kulawiak, B., and Szewczyk, A. (2022). Current challenges of mitochondrial potassium channel research. Front. Physiol. 13, 907015. doi:10.3389/fphys.2022.907015
Kulawiak, B., Żochowska, M., Bednarczyk, P., Galuba, A., Stroud, D. A., and Szewczyk, A. (2023). Loss of the large conductance calcium-activated potassium channel causes an increase in mitochondrial reactive oxygen species in glioblastoma cells. Pflugers Arch. 475, 1045–1060. doi:10.1007/s00424-023-02833-9
Laskowski, M., Augustynek, B., Bednarczyk, P., Żochowska, M., Kalisz, J., O'Rourke, B., et al. (2019). Single-Channel properties of the ROMK-pore-forming subunit of the mitochondrial ATP-sensitive potassium channel. Int. J. Mol. Sci. 20, 5323. doi:10.3390/ijms20215323
Laskowski, M., Kicinska, A., Szewczyk, A., and Jarmuszkiewicz, W. (2015). Mitochondrial large-conductance potassium channel from Dictyostelium discoideum. Int. J. Biochem. Cell Biol. 60, 167–175. doi:10.1016/j.biocel.2015.01.006
Leanza, L., Checchetto, V., Biasutto, L., Rossa, A., Costa, R., Bachmann, M., et al. (2019). Pharmacological modulation of mitochondrial ion channels. Br. J. Pharmacol. 176 (22), 4258–4283. doi:10.1111/bph.14544
Leanza, L., Zoratti, M., Gulbins, E., and Szabo, I. (2014). Mitochondrial ion channels as oncological targets. Oncogene 33, 5569–5581. doi:10.1038/onc.2013.578
Lewandowska, J., Kalenik, B., Wrzosek, A., and Szewczyk, A. (2024). Redox regulation of mitochondrial potassium channels activity. Antioxidants 13, 434. doi:10.3390/antiox13040434
Liu, M., and Dudley, S. C. (2020). Magnesium, oxidative stress, inflammation, and cardiovascular disease. Antioxidants 9, 907. doi:10.3390/antiox9100907
Liu, Y., Sato, T., Seharaseyon, J., Szewczyk, A., O'Rourke, B., and Marbán, E. (1999). Mitochondrial ATP-dependent potassium channels: viable candidate effectors of ischemic preconditioninga. Ann. N. Y. Acad. Sci. 874, 27–37. doi:10.1111/j.1749-6632.1999.tb09222.x
Lukowski, R., Cruz Santos, M., Kuret, A., and Ruth, P. (2022). cGMP and mitochondrial K+ channels-Compartmentalized but closely connected in cardioprotection. Br. J. Pharmacol. 179, 2344–2360. doi:10.1111/bph.15536
Matkovic, K., Koszela-Piotrowska, I., Jarmuszkiewicz, W., and Szewczyk, A. (2011). Ion conductance pathways in potato tuber (Solanum tuberosum) inner mitochondrial membrane. Biochim. Biophys. Acta. 1807, 275–285. doi:10.1016/j.bbabio.2010.12.001
Mitchell, P. (1985). The correlation of chemical and osmotic forces in biochemistry. J. Biochem. 97, 1–18. doi:10.1093/oxfordjournals.jbchem.a135033
Olszewska, A., and Szewczyk, A. (2013). Mitochondria as a pharmacological target: Magnum overview. IUBMB Life 65, 273–281. doi:10.1002/iub.1147
O'Rourke, B. (2007). Mitochondrial ion channels. Annu. Rev. Physiol. 69, 19–49. doi:10.1146/annurev.physiol.69.031905.163804
Paggio, A., Checchetto, V., Campo, A., Menabò, R., Di Marco, G., Di Lisa, F., et al. (2019). Identification of an ATP-sensitive potassium channel in mitochondria. Nature 572, 609–613. doi:10.1038/s41586-019-1498-3
Piwonska, M., Wilczek, E., Szewczyk, A., and Wilczynski, G. M. (2008). Differential distribution of Ca2+-activated potassium channel beta4 subunit in rat brain: Immunolocalization in neuronal mitochondria. Neuroscience 153, 446–460. doi:10.1016/j.neuroscience.2008.01.050
Severin, F., Urbani, A., Varanita, T., Bachmann, M., Azzolini, M., Martini, V., et al. (2022). Pharmacological modulation of Kv1.3 potassium channel selectively triggers pathological B lymphocyte apoptosis in vivo in a genetic CLL model. J. Exp. Clin. cancer Res. 41 (1), 64. doi:10.1186/s13046-022-02249-w
Singh, H., Lu, R., Bopassa, J. C., Meredith, A. L., Stefani, E., and Toro, L. (2013). MitoBK(Ca) is encoded by the Kcnma1 gene, and a splicing sequence defines its mitochondrial location. Proc. Natl. Acad. Sci. U. S. A. 110, 10836–10841. doi:10.1073/pnas.1302028110
Singh, H., Stefani, E., and Toro, L. (2012). Intracellular BK(Ca) (iBK(Ca)) channels. J. Physiology 590, 5937–5947. doi:10.1113/jphysiol.2011.215533
Sorgato, M. C., Keller, B. U., and Stühmer, W. (1987). Patch-clamping of the inner mitochondrial membrane reveals a voltage-dependent ion channel. Nature 330, 498–500. doi:10.1038/330498a0
Szabo, I., and Szewczyk, A. (2023). Mitochondrial ion channels. Annu. Rev. Biophys. 52, 229–254. doi:10.1146/annurev-biophys-092622-094853
Szabo, I., and Zoratti, M. (2014). Mitochondrial channels: Ion fluxes and more. Physiol. Rev. 94, 519–608. doi:10.1152/physrev.00021.2013
Szewczyk, A. (1996). The ATP-regulated K+ channel in mitochondria: Five years after its discovery. Acta Biochim. Pol. 43, 713–719. doi:10.18388/abp.1996_4469
Szewczyk, A., and Bednarczyk, P. (2018). Modulation of the mitochondrial potassium channel activity by infrared light. Biophysical J. 114, 43a. doi:10.1016/j.bpj.2017.11.288
Szewczyk, A., Jarmuszkiewicz, W., and Kunz, W. S. (2009). Mitochondrial potassium channels. IUBMB Life 61, 134–143. doi:10.1002/iub.155
Szewczyk, A., Kajma, A., Malinska, D., Wrzosek, A., Bednarczyk, P., Zabłocka, B., et al. (2010). Pharmacology of mitochondrial potassium channels: Dark side of the field. FEBS Lett. 584, 2063–2069. doi:10.1016/j.febslet.2010.02.048
Szewczyk, A., and Marbán, E. (1999). Mitochondria: A new target for K channel openers? Trends Pharmacol. Sci. 20, 157–161. doi:10.1016/s0165-6147(99)01301-2
Szewczyk, A., Skalska, J., Głab, M., Kulawiak, B., Malińska, D., Koszela-Piotrowska, I., et al. (2006). Mitochondrial potassium channels: From pharmacology to function. Biochim. Biophys. Acta. 1757, 715–720. doi:10.1016/j.bbabio.2006.05.002
Szewczyk, A., and Wojtczak, L. (2002). Mitochondria as a pharmacological target. Pharmacol. Rev. 54, 101–127. doi:10.1124/pr.54.1.101
Szteyn, K., and Singh, H. (2020). BKCa channels as targets for cardioprotection. Antioxidants 9, 760. doi:10.3390/antiox9080760
Testai, L., Rapposelli, S., Martelli, A., Breschi, M. C., and Calderone, V. (2015). Mitochondrial potassium channels as pharmacological target for cardioprotective drugs. Med. Res. Rev. 35, 520–553. doi:10.1002/med.21332
Walewska, A., Krajewska, M., Stefanowska, A., Buta, A., Bilewicz, R., Krysiński, P., et al. (2022). Methods of measuring mitochondrial potassium channels: a critical assessment. Int. J. Mol. Sci. 23, 1210. doi:10.3390/ijms23031210
Walewska, A., Szewczyk, A., and Koprowski, P. (2018). Gas signaling molecules and mitochondrial potassium channels. Int. J. Mol. Sci. 19, 3227. doi:10.3390/ijms19103227
Wrzosek, A., Augustynek, B., Żochowska, M., and Szewczyk, A. (2020). Mitochondrial potassium channels as druggable targets. Biomolecules 10, 1200. doi:10.3390/biom10081200
Wrzosek, A., Gałecka, S., Żochowska, M., Olszewska, A., and Kulawiak, B. (2022). Alternative targets for modulators of mitochondrial potassium channels. Molecules 27, 299. doi:10.3390/molecules27010299
Xu, W., Liu, Y., Wang, S., McDonald, T., Van Eyk, J. E., Sidor, A., et al. (2002). Cytoprotective role of Ca2+- activated K+ channels in the cardiac inner mitochondrial membrane. Science 298, 1029–1033. doi:10.1126/science.1074360
Keywords: mitochondria, potassium channels, reactive oxygen species, cytoprotection, potassium channel openers
Citation: Szewczyk A (2024) Understanding mitochondrial potassium channels: 33 years after discovery. Acta Biochim. Pol 71:13126. doi: 10.3389/abp.2024.13126
Received: 13 April 2024; Accepted: 20 May 2024;
Published: 28 May 2024.
Edited by:
Grzegorz Wegrzyn, University of Gdansk, PolandReviewed by:
Agata Wawrzkiewicz-Jałowiecka, Silesian University of Technology, PolandMartin Jabůrek, Academy of Sciences of the Czech Republic (ASCR), Czechia
Dominika Malińska, Polish Academy of Sciences, Poland
Copyright © 2024 Szewczyk. This is an open-access article distributed under the terms of the Creative Commons Attribution License (CC BY). The use, distribution or reproduction in other forums is permitted, provided the original author(s) and the copyright owner(s) are credited and that the original publication in this journal is cited, in accordance with accepted academic practice. No use, distribution or reproduction is permitted which does not comply with these terms.
*Correspondence: Adam Szewczyk, YS5zemV3Y3p5a0BuZW5ja2kuZWR1LnBs