- 1Department of Pathology and Immunology, Baylor College of Medicine, Houston, TX, United States
- 2Department of Neuroscience, Baylor College of Medicine, Houston, TX, United States
- 3Jan and Dan Duncan Neurological Research Institute at Texas Children’s Hospital, Houston, TX, United States
- 4Department of Pediatrics, Baylor College of Medicine, Houston, TX, United States
- 5Development, Disease Models and Therapeutics Graduate Program, Baylor College of Medicine, Houston, TX, United States
Dystonia is the third most common movement disorder. It causes debilitating twisting postures that are accompanied by repetitive and sometimes intermittent co- or over-contractions of agonist and antagonist muscles. Historically diagnosed as a basal ganglia disorder, dystonia is increasingly considered a network disorder involving various brain regions including the cerebellum. In certain etiologies of dystonia, aberrant motor activity is generated in the cerebellum and the abnormal signals then propagate through a “dystonia circuit” that includes the thalamus, basal ganglia, and cerebral cortex. Importantly, it has been reported that non-motor defects can accompany the motor symptoms; while their severity is not always correlated, it is hypothesized that common pathways may nevertheless be disrupted. In particular, circadian dysfunction and disordered sleep are common non-motor patient complaints in dystonia. Given recent evidence suggesting that the cerebellum contains a circadian oscillator, displays sleep-stage-specific neuronal activity, and sends robust long-range projections to several subcortical regions involved in circadian rhythm regulation, disordered sleep in dystonia may result from cerebellum-mediated dysfunction of the dystonia circuit. Here, we review the evidence linking dystonia, cerebellar network dysfunction, and cerebellar involvement in sleep. Together, these ideas may form the basis for the development of improved pharmacological and surgical interventions that could take advantage of cerebellar circuitry to restore normal motor function as well as non-motor (sleep) behaviors in dystonia.
Introduction
Dystonia is a neurological disease that is currently considered the third most common movement disorder. It is characterized by co-contractions and/or over-contractions of agonist and antagonist muscles. These changes in muscle function result in frequent, often painful, paroxysms of twisting postures, though in rare cases dystonia can manifest as a sustained dystonic crisis. The etiology of dystonia remains incompletely understood, in part because “dystonia” does not comprise a singular disorder, but rather it describes the observed phenotype, or “phenomenology”, of an array of motor disorders that produce a shared clinical presentation (1). While dystonia can arise from a growing list of inherited genetic mutations (e.g., DYT1/TOR1A dystonia), as a comorbidity in other neurological disorders such as Parkinson’s disease, tremor and ataxia, or as a result of traumatic brain injury, most diagnoses of dystonia are in fact idiopathic (2).
Historically, dystonia was considered a basal ganglia disease (3), and indeed therapeutic electrical stimulation of basal ganglia structures is successfully used to treat some, often primary, dystonia patients (4). More recently, however, dystonia has been re-characterized as a network disorder (5–8), implicating an array of brain regions, chief among them being the cerebellum. Studies in both human patients (5) and rodent models (9) have suggested that the cerebellum plays a key role in the onset and regulation of dystonia. Indeed, genetic, molecular, and pharmacological manipulation of cerebellar activity in several animal models has been shown to cause robust and severe dystonic phenotypes (9–12). Among these phenotypes are the altered muscle contractions and consequent abnormal movements such as twisting postures, hyper-extended limbs, and tremor.
As might be expected from a network disorder, the characteristic motor symptoms of dystonia can present alongside various non-motor symptoms, including impaired sleep (13). It is established that the cerebellum expresses clock genes and contains a circadian oscillator (14), and that sleep plays a key role in the maintenance of normal cerebello-thalamo-cortical coherence (15). Yet, the causality of this relationship between circadian centers, the cerebellum, and dystonia remains largely unknown. Therefore, in this review, we discuss research on each of these topics in order to highlight key relationships, which we argue merit further experimental and clinical investigation. In particular, we not only explore the evidence supporting a role for the cerebellum in dystonic movements but also its potential involvement in non-motor behavioral disturbances in dystonia.
A Primer on Cerebellar Cellular Composition and Circuitry
The cerebellar circuit is well-characterized but warrants a brief overview to help contextualize the forthcoming discussion. The broad organization of the cerebellum includes the cerebellar cortex, which receives afferent input from the central nervous system (CNS) and peripheral nervous system (PNS). Major afferent systems that terminate in the cerebellar cortex include those that originate from the pontine nuclei, vestibular nuclei, inferior olive, and spinal cord. The cortex surrounds the cerebellar nuclei, which receive projections from the cerebellar cortex. The cerebellar nuclei also receive collateral projections from the inferior olive, pons, reticular nuclei, vestibular nuclei and also from the spinal cord, among other regions.
The cerebellar cortex is organized into three distinct layers: the molecular layer, the Purkinje cell layer, and the granular layer. The outermost layer, the molecular layer, contains the dendrites of Purkinje cells, which receive an impressive amount of synaptic input. In rodents, each Purkinje cell receives more than 200,000 inputs. The vast majority of these inputs arise from the axons of granule cells, called parallel fibers, which form excitatory synapses on the Purkinje cell dendritic spines (16). Purkinje cells receive additional innervation from stellate cell and Basket cell interneurons, which both provide inhibitory signals onto the Purkinje cells (17). Finally, Purkinje cell dendrites in the molecular layer also receive direct and powerful excitatory input from climbing fibers, which originate in the inferior olive and “climb” through the Purkinje cell dendrites eventually innervating the shafts of the dendritic branches. Climbing fiber signals are thought to play a critical role in error signaling and motor learning (18). More recently, behavioral paradigms combined with optogenetics manipulations suggest a role for climbing fibers in reward prediction (19–21).
Purkinje cells bodies constitute the middle of the three layers, in the aptly named Purkinje cell layer. The Purkinje cell soma and axon initial segment receive inhibitory input from the Basket cells. The Purkinje cell layer also contains the somata of Bergman glia and candelabrum cells (22). Importantly, despite the variety of cell types present, the Purkinje cell layer is in fact a monolayer.
The innermost layer of the cerebellar cortex, the granular layer, contains the somata of the cerebellar granule cells. Granule cells are the most numerous neuron type in the brain (23). The granule cells receive mossy fiber input, representing afferents from diverse regions, including the pontine and vestibular nuclei, the spinal cord, and the reticular nuclei, along with inhibitory input from Golgi cells, a local cerebellar interneuron (24). A subset of granule cells also receives excitatory input from a specialized class of signal-amplifying interneurons called unipolar brush cells (25).
Purkinje cells, which receive inputs from the various excitatory and inhibitory afferents, are the sole output of the cerebellar cortex and project to the inner core of the cerebellum, terminating upon the cerebellar nuclei. Representing the primary output from the cerebellum, the cerebellar nuclei are divided into four distinct structures in humans: dentate, emboliform, globose, and fastigial. The emboliform and globose are referred to as the interposed nuclei in rodents, in which the distinction between the sub-nuclei is less clear (26). The dentate is the most lateral and receives the majority of its inputs from the cerebellar hemispheres. The interposed nuclei are located in the middle and receive input from the paravermis. The most medial nuclei, the fastigial, receive input predominantly from the vermis. While the Purkinje cell to cerebellar nuclei connection is strictly inhibitory, cerebellar nuclei efferent signals are multimodal as they contain long-range glutamatergic and GABAergic projection neurons, as well as local glycinergic interneurons (24). It should be noted that although they are not located within the cerebellum, the vestibular nuclei, located directly below the cerebellum, also receive input from Purkinje cells.
The Cerebellum in Dystonia
The etiology of dystonia is complex. While the emergence of dystonia likely involves the dysfunction of a broad but convergent dystonia circuit, it is possible that hereditary forms of dystonia with a defined molecular origin involve distinct subcomponents of this circuit. DYT1 generalized dystonia, a common form of hereditary dystonia, can arise from a 3-base-pair deletion (∆GAG) in TOR1A. TOR1A encodes torsinA, a cytoplasmic protein found at high concentrations in Purkinje cells and the dentate nucleus (27). This mutation is known to result in aberrant binding activity between torsinA and its partners (28) and impaired torsin-ATPase activation (29). Ultimately, this mutation is known to affect neurodevelopment through disrupted formation of the nuclear envelope (30). It is also understood that abnormal torsinA activity in the cerebellum is directly related to the development of dystonia: shRNA knockdown of torsinA in adult mice results in dystonia only when delivered to the cerebellum, but not the basal ganglia (31). This suggests that while the characterization of pathways for torsinA activity in dystonia are still underway, the cerebellum appears to be a key node in the network of disrupted brain regions. This relationship holds for other hereditary dystonias. Mutations in THAP1, a transcriptional regulator of endothelial cell proliferation, are known to cause DYT6 dystonia (12). Thap1+/− mutant mice display distinct cerebellar abnormalities, including a lower frequency of cerebellar nuclei firing and Purkinje cells that fire with greater regularity than littermate controls (32). Similarly, Sgce and ATP1α3 mutant mice (models for DYT11 and DYT12 dystonia respectively) also display aberrant Purkinje cell and cerebellar nuclei firing patterns (33, 34). While the understanding of how these disease-causing mutations result in altered cerebellar circuit function and subsequent dystonia is unclear, some work does suggest that these genes converge on the eIF2α signaling pathway. Responsible for catalyzing protein synthesis and processing in the endoplasmic reticulum (35), mutations in this pathway may result in endoplasmic reticulum stress, which increases Ca2+ permeability and alters neuronal Ca2+ signaling (36).
The cerebellum’s role in non-hereditary dystonia etiology is also elaborate. In idiopathic cervical dystonia, fMRI studies have demonstrated that lesions across the brain comprise a functionally connected network, dubbed the “dystonia network,” characterized by positive connectivity to the cerebellum (5). Independent groups have found that botulinum neurotoxin (BoNT) injections, now a standard, efficacious therapy for many dystonia patients (2), can result in decreased intra-cerebellar connectivity in the posterior cerebellum and decreased cerebro-cerebellar connectivity between the cerebellar vermis and the frontal/prefrontal cortices (37). Such studies suggest that overactivity of the cerebellum contributes to the development of dystonia-related behaviors. Importantly, cerebellar overactivity may serve as a “marker” for specific forms of dystonia, as studies in patients with Parkinson’s Disease show similar results to fMRI studies in patients with cervical dystonia; both groups display characteristic cerebellar overactivity (5, 38). Therefore, further imaging studies may be able to shed light on whether cerebellar dysfunction is distinct in different manifestations of the disease, or whether cerebellar overactivity is a consistent hallmark in subsets of dystonia.
While both molecular evidence from hereditary dystonia studies and circuit level evidence from human imaging implicate functional cerebellar alterations in dystonia, the manner in which aberrant cerebellar circuit function produces dystonic movement remains enigmatic. To better understand this relationship, a brief description of putative cerebellar computational models may be helpful. An interesting observation is that up to 98% of neurotypical Purkinje cell-parallel fiber synapses may be functionally silent with the synaptic signal at any given parallel fiber-Purkinje cell synapse having only a negligible effect on the postsynaptic cell (39–41). It has been proposed that this is a deliberate process which maximizes the signal to noise ratio of convergent parallel fiber inputs allowing for adequate signal processing, by the Purkinje cell, ultimately leading to the production of appropriate voluntary movement (42). Such a model assumes a covariance learning rule in which parallel fiber synapses with less noise have an increased synaptic weight, and vice versa. Thus, this model posits the cerebellum as an adaptive computational network (43), centered upon Purkinje cell signal processing. Together with the dystonia network hypothesis, these circuit-level features may support the notion that aberrant overactivity in the cerebellum, particularly impaired Purkinje cell sorting ability, could be a major contributor to dystonia pathophysiology. Although it remains unclear how these inputs and cortical stages of signal processing in the cerebellum are affected in various etiologies of dystonia, there is mounting evidence that dystonia converges on the cerebellar nuclei and as a result severely disrupts cerebellar functional output and behavior.
In addition to the aforementioned findings, several mouse models of dystonia indeed suggest that cerebellar output function may be the key to dystonia pathophysiology. One study demonstrated that infusion of ouabain at the cerebellar midline leads to altered cerebellar activity and the resultant dystonia, the severity of which can then be lessened by cerebellar infusion of GABA (44). Concordantly, the tottering mouse possesses a mutation in P/Q-type calcium channels expressed in cerebellar granule cells and Purkinje cells. When crossed to a mutant mouse line with Purkinje cell degeneration, the dystonia disappears (9). Loss of Purkinje cell afferent function also contributes to dystonia pathophysiology. The Ptf1aCre;Vglut2fx/fx mouse, in which excitatory olivocerebellar synapses are silenced, experiences severe dystonic attacks and displays aberrant Purkinje cell function and burst-like cerebellar nuclei firing patterns. Importantly, in this mouse, mobility is restored by modulating the cerebellar circuit with deep brain stimulation directed to the cerebellar nuclei (10). Models of hereditary dystonia also implicate cerebellar nuclei dysfunction as a key component of their etiology. The Tor1aΔE mouse, a model for DYT1 dystonia, presents with significant glial fibrillary acidic protein staining in the cerebellar nuclei (45), which is indicative of reactive astrocytes and suggestive of significant sustained damage in this region. Overexpression of torsinB (a paralog of torsinA, and which is also expressed in Purkinje cells), is able to rescue the main motor phenotypes as well as the accompanying degeneration and cerebellar gliosis (45).
The findings that the hereditary dystonias, the effective treatments of idiopathic dystonias, and the circuit manipulations that produce dystonic behaviors in model systems all converge to implicate altered cerebellar network function in disease pathophysiology begs an important question: is dystonia truly an isolated motor disorder or are the observed neurological deficits the reflection of primary manifestations of disease that affect a broader brain network? To start to address this question, in the next section, the non-motor symptoms of dystonia will be further explored. Importantly, we will do so in the context of the expanding roles of the cerebellum.
The Cerebellum and the Non-Motor Symptoms of Dystonia
As a network disorder, it is expected that the aberrant cerebellar activity leading to dystonia should, in theory, impact all regions with cerebellar connectivity, which would then affect diverse physiological processes. To wit, alongside motor dysfunction, patients with dystonia often present with a variety of non-motor symptoms; depression, anxiety, and disordered sleep being common among them (46–48). Given the debilitating nature of the motor phenotypes seen in dystonia, it may seem reasonable to assume, and often is, that non-motor symptoms are a secondary result of motor dysfunction. Indeed, dystonia patients who experience painful and involuntary motor contractions can often also experience depression, anxiety, and disrupted sleep as a direct result of their motor symptoms. However, various lines of evidence suggest that these non-motor symptoms are independent manifestations of the network dysfunction that leads to dystonia. While some patients do experience improvements in depression following BoNT treatment targeting motor symptoms (49), others see little improvement, failing to see any causative relationship between motor symptom severity and non-motor symptoms (50). Impaired sleep, in particular, appears to be unaffected by BoNT therapy (51, 52). These results are in line with research delineating the role of the cerebellum in regulating non-motor behaviors (53–56), suggesting that the non-motor symptoms are a result of aberrant cerebellar activity in dystonia, rather than a result of the ultimate motor deficits generated by a canonical network. These findings are also intriguing as they suggest a differential role for cerebellar hyperconnectivity versus hyperactivity in the disease. While reduced cerebellar hyperconnectivity is achieved by BoNT therapy (37), it is possible that cerebellar hyperactivity is the greater contributor to sleep impairments in dystonia, resulting in the observed inability of BoNT therapy to improve sleep. Alternatively, it may be that the degree of cerebellar quiescence achieved by focal BoNT therapy is insufficient to restore sleep, which involves highly complex processes.
It is also possible that non-motor symptoms in dystonia, like sleep disturbances, do not depend solely on cerebellar dysfunction but rather stem from aberrant bi-directional coupling between the cerebellum and cerebral cortex. For instance, activity in the cerebral cortex is known to down-regulate cerebellar output activity during slow-wave (NREM) sleep (57); yet cerebellar activity is also proposed to fine tune neocortical synchrony during NREM sleep (58). As dystonia is known to disrupt communication between the cerebellum and cortical structures (5), it may be that aberrant cerebello-cortical signaling drives the non-motor symptoms of dystonia, such as impaired sleep.
Sleep impairments in dystonia are particularly notable, although their causes are unclear. It is well-documented that patients with dystonia typically present with insomnia or some form of sleep disruption (51, 59–61). For instance, polysomnographic recordings conducted in cervical dystonia patients have shown that these individuals display an increased latency to sleep, increased latency to REM, and decreased sleep efficiency (62). However, sleep impairments in dystonia are particularly understudied, due in part to perceptions that they represent a secondary symptom of the disease rather than an integral and distinct etiological component, a view that is gradually changing (51).
Circadian Rhythms and Sleep
Circadian rhythms represent the output of many biological clocks that are distributed throughout the brain and entire body (Figure 1). The suprachiasmatic nucleus (SCN), a relatively small nucleus consisting of ∼20,000 neurons in the hypothalamus, acts as the principal circadian clock (63). The time-keeping ability of the SCN is maintained by a transcription-translation feedback loop; the rhythmic activation and repression of Bmal1, Clock, Per and Cry comprise the core components of this molecular feedback loop, which has a period of approximately 24-h (64). Although this remarkable time keeping ability is endogenously generated, it must be “reset” each day to maintain fidelity to a 24-h cycle. Therefore, these clocks rely on rhythmically occurring environmental factors, “zeitgebers”, to provide regulatory cues (63). While circadian clocks are capable of entraining to a variety of zeitgebers, including mealtimes (65), temperature (66), and circulating glucocorticoids (i.e., cortisol) (67, 68), the primary mammalian zeitgeber remains the light-dark cycle. This presents a fundamental link between an animal’s behavior and brain circuitry, as the SCN receives direct retinal innervation through the retino-hypothalamic tract (63).
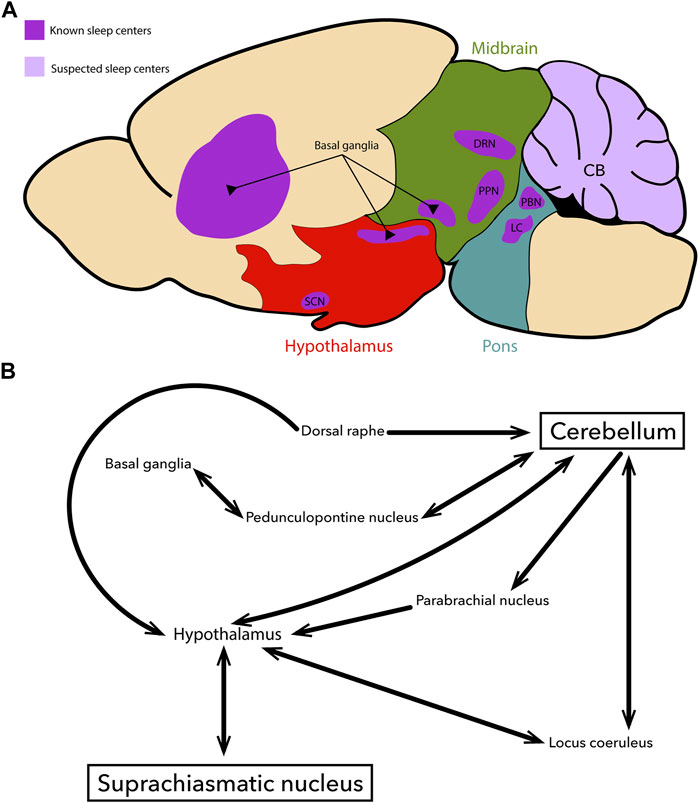
FIGURE 1. Overview schematic of key brain regions controlling sleep and circadian rhythms (A) An illustration of a sagittal section cut through the adult mouse brain. The pons (blue), midbrain (green), and hypothalamus (red) are highlighted to designate their relative positions. Regions that are known to participate in the regulation of sleep-related processes are shown in dark purple. Regions which are suspected to participate in sleep regulation are shown in light purple. All regions of interest send or receive inputs to/from the cerebellum, the suprachiasmatic nucleus, or the hypothalamus. Although the image here depicts circuits related to the main circadian sleep centers, there are also other circuits that contribute to controlling neural function during sleep. (B) An overview circuit schematic depicting the major neural projections between key regions that are involved in sleep physiology (A). For simplicity, other regions (for example the thalamus) and their related circuits were excluded, although we fully acknowledge their importance in sleep.
Communicating with a number of “peripheral clocks” (non-SCN clocks) via autonomic innervation and humoral signals (69), the SCN orchestrates the timing of varied physiological functions, including sleep. Indeed, ablation of the SCN results in the inability to entrain to photic and non-photic stimuli (70), and complete arrhythmicity in daily patterns of activity and sleep timing (71). Typically, circadian disruption in healthy individuals stems from environmental factors or pre-existing conditions exacerbating circadian dysfunction. Shift work is well-known to cause significant circadian misalignment and subsequent comorbidities ranging from mood and social disorders (72, 73), to compromised immune function (74, 75) or cardiovascular disease (72, 76, 77). Evidence also exists that chronic short sleep directly impacts mechanisms of neurodegeneration, observed in its contribution to the pathophysiology of Alzheimer’s disease via disruption of glymphatic clearance (78–80). Sleep impairments are also highly associated with motor learning/function (81–83). Eye-blink conditioning experiments in mice demonstrate a significant tendency towards more conditioned responses when mice sleep longer immediately following learning trials (84). Similar results were observed in humans performing a cued motor task, in which replaying the auditory cue during NREM sleep improved performance in the trial immediately following the sleep bout (85). Memory consolidation during sleep also serves an important role in the recovery of motor function following injury. Patients in recovery from ischemic stroke display improved retention of implicit motor learning when they are able to sleep between practice and testing periods (86).
While more experimental work is needed in this area, several lines of evidence do exist to suggest a causal link between cerebellar damage in dystonia and sleep dysfunction. Both climbing fibers and mossy fibers, which represent the major afferent pathways to the cerebellum from diverse brain regions, demonstrate differential activity during specific sleep stages (87), and their altered activity is implicated in different models of dystonia (10, 88). On the cerebellar output side of the structure, lesions of the superior cerebellar peduncle (a large collection of tracts mainly carrying the primary output pathways of the cerebellum) and the cerebellar vermis result in reductions of NREM and REM sleep in cats (89, 90). Therefore, perhaps the peripheral clocks, in particular the cerebellum, may play a causal role in the generation of disrupted sleep-associated comorbidities, as well as direct disturbances of sleep, as we will discuss in the next section.
The Cerebellum, Connectivity and Sleep Physiology
Similarly to other structures containing peripheral clocks [i.e., dorsomedial hypothalamus (91), liver (92)] the cerebellum also harbors a circadian oscillator (Figure 1B). Evidence exists not only for rhythmic expression of cerebellar clock genes and proteins, but also that this cerebellar oscillator plays an active role in regulating physiological behaviors, namely food-anticipatory activity, as mealtime anticipation behaviors are lacking in mice with cerebellar damage (14). The cerebellar oscillator may also be sensitive to corticosterone, a hormone which is known to oscillate with a distinct circadian profile (67), as SCN-lesioned rats displayed restored cerebellar clock gene rhythms when they received exogenous corticosterone. Interestingly, exogenous corticosterone administration and subsequent restoration of the cerebellar oscillator failed to restore disruption of body temperature and running wheel activity (93). This apparent specificity in the restoration of only some circadian behaviors could suggest that the cerebellar oscillator acts in partial independence from core circadian clocks, or only selectively regulates certain circadian behaviors. In rats, the thyroid hormone, triiodothyronine, also appears to exert circadian regulatory effects on the cerebellar oscillator, although these effects are limited to the neonatal developmental stage (94).
While direct anatomical connections between the SCN and cerebellum are lacking, evidence for indirect projections via intermediary regions does exist (boxed regions in Figure 1B) (95). Such connections are critical, as peripheral oscillators must receive phase-setting information from the SCN, even if indirectly. The locus coeruleus may serve as such an intermediary region. Not only does it directly regulate sleep-wake transitions (96) and NREM/REM intensity (97) through monoaminergic neurons, it also sends direct projections to the cerebellum and exerts a modulatory effect on Purkinje cells (98–100). Notably, both Purkinje cells and the deep cerebellar nuclei send dense projections to the locus coeruleus (LC); Purkinje cell projections in particular represent one of the biggest fractional inputs (101). This intermediary region is particularly notable, as the LC may influence cerebellar-dependent learning/plasticity in much the same way as it is known to affect place cell dependent hippocampal activity (97).
The brainstem may also enable, or perhaps facilitate, cerebellar-circadian communication. The parabrachial nucleus in the rostral hindbrain projects directly to the hypothalamus (102) and is known to play a role in REM-NREM transitions (103, 104) while also receiving input from Purkinje cells in lobules VIII–X of the cerebellum (105). The serotonergic neurons of the dorsal raphe nucleus are also well known regulators of sleep-wake states (106–108) and have recently been shown to play a role in mediating stress-induced dystonia through direct projections to the cerebellar nuclei (109). Additionally, the pedunculopontine nucleus sends direct projections to the SCN (110) and is established as a regulator of REM sleep (111–113) while also sending and receiving inputs between the cerebellum and the basal ganglia (111, 114). Cholinergic abnormalities in the pedunculopontine nucleus (PPN) have also been observed in patients with cervical dystonia, further suggesting that it may contribute to the neural circuitry that is defective in dystonia, although the exact etiology and the mechanistic underpinnings of how this structure and its connectivity contributes to the disease are unclear (115). It is known that cholinergic signaling directly modulates arousal states, and that cholinergic signaling of the PPN, in particular, increases wake and REM states, and also plays a key role in the initiation of REM sleep (116). In this way, a cholinergic deficiency in the PPN could directly contribute to both cerebellar and sleep dysfunctions, and given the known recurrent activity between the two regions (5), aberrant activity of the PPN and cerebellum in dystonia may ultimately compound one another to impact the same behavioral disturbances.
The dopaminergic neurons of the ventral tegmental area (VTA) may also serve as an intermediary region allowing for the cerebellum to participate in sleep regulation. Dopaminergic VTA neurons promote arousal, and their suppression is known to inhibit wakefulness while also promoting sleep-relevant behaviors (117). Cerebellar neurons including Purkinje cells are also reported to receive dopaminergic input and express dopamine receptors (118), while the VTA itself receives afferents from the cerebellum directly (58). In this way, the VTA may act as a key intermediary in the nonmotor symptoms of dystonia, particularly in dopa-responsive dystonia (119).
Perhaps one of the most functionally provocative but poorly studied intermediary circuits is between the cerebellum and hypothalamus. Hypothalamo-cerebellar projections are widespread and comprise a topographic organization. Projections originate primarily in the posterior hypothalamus and terminate in all divisions of the cerebellar nuclei cells as well as within the Purkinje cell and granule cell layers in the cerebellar cortex, with a heavy projection to the vermis. The cerebellar nuclei in turn send reciprocal connections to the hypothalamus, again with a topographic organization, projecting through the superior cerebellar peduncle (120). It is interesting to note that the SCN has significant reciprocal connections with the posterior hypothalamus (121). These reciprocal connections, whose non-canonical nature results in their escape from regular descriptions of the cerebellar circuit, could provide at least one potential route for communication between the cerebellum and the SCN.
Alongside these neuroanatomical relationships, there is also evidence to suggest that the cerebellum plays a role in sleep regulation. Purkinje cells have been demonstrated to express sleep-stage dependent activity, increasing their firing in sleep-wake transitions (55). It should be noted that the exact changes in firing dynamics during REM/NREM are conflicting: some have shown that Purkinje and cerebellar nuclei cell activity increases during REM (122, 123) while others show the opposite (55). The latter is appealing given the role of Purkinje cells in modulating muscle tone (124), which is characteristically atonic during REM sleep (125). fMRI studies during sleep have also demonstrated that the cerebellum displays increased activity during NREM sleep, which may facilitate neuronal interactions (126). Indeed, this increase in cerebellar activity is in agreement with hypotheses that motor learning is refined during sleep (82–85), and with recent findings that delta wave oscillations during NREM sleep are responsible for coordinating cerebello-hippocampal temporal coherence (127). In line with this, recent work also suggests that cerebello-thalamo-cortical coherence is increased during REM sleep (15), indicative of an offline mechanism for learning consolidation. These cycles of increased and decreased coherence track with changing sleep stages, suggesting that there are periods during which cerebellar “access” to thalamic and cortical structures is preferentially optimized. While the ultimate goal of this optimized coherence is hypothesized to involve predictive learning, which is consistent with the model of cerebellar feedforward control mechanisms (128), further work is needed to fully understand not only the properties of cerebellar activity during sleep, but also its role in sleep regulation.
Cerebellar activity during sleep also plays an important role in normal sensorimotor development. While REM sleep is characteristically defined by muscle atonia (125), skeletal muscle twitches are known to occur in mammals, particularly in rodents. These twitches are implicated in normal somatotopic organization, and are believed to play a role in the maturation of movement patterns, as rudimentary movements in early development become more coordinated, adaptive movements in adulthood (129). Cerebellar development is thought to be linked to both twitch-related activity and REM sleep. Previous work has demonstrated that 6-day old rats (in which the parallel fiber system is not yet fully formed) display significant state-dependent changes in complex spike and simple spike activity. Complex and simple spike activity increases during REM sleep, and increase yet again during bouts of twitch activity occurring in REM sleep (130). As such, twitches may act as a source of activity that contributes to the development of cerebellar sensorimotor regulation (131). Similar work in 4, 8, and 12-day old rats found REM-dependent increases in Purkinje cell activity (i.e., increased complex spikes and simple spikes), which peaks at 8 days of age and is temporally aligned with muscle twitches, to within 100 ms (132). Recently, head-fixed sleep recordings in older rats (9, 12, and 20-days of age) suggest that twitches occurring in REM sleep trigger somatotopic activity in the thalamus and motor cortex, and that twitch-related activity in the ventrolateral and ventral-posterior thalamus undergoes continued refinement from P12 to P20. Of particular interest is the finding that this forward model of movement in the ventrolateral thalamus is cerebellar dependent, as pharmacokinetic inactivation of the interposed nucleus disrupts the precise timing of twitch-related activity that has been established by P20 (133). Such work establishes the role of specific movements, such as twitches during sleep, in training and calibrating internal models, which allow for the decoding of motor and sensory information during a key developmental window.
Thus, the cerebellum may be considered a central physiologic component in sleep, acting as a peripheral clock whose activity appears to play a significant role in the regulation of normal sleep homeostasis. However, a major question still remains: what are the mechanistic effects of disrupted cerebellar function on sleep? As we have alluded to previously, evidence for such a causal relationship does exist at both the functional and circuit levels (87, 89, 90). In this next section, we will explore this relationship, specifically in the context of dystonia-related abnormalities.
Possible Mechanistic Interactions Between the Cerebellum, Sleep, and Dystonia
Dystonia, as a network disorder, may be considered at the behavioral level as the outcome of cerebellar overactivity (2, 5). Indeed, although BoNT treatment impacts neuromuscular function, it may also decrease cerebellar connectivity between the ipsilateral posterior vermis and the posterior hemispheres in patients with cervical dystonia (37). This decreased connectivity extends outside the cerebellum, impacting cerebello-cortical projections from the vermis/crus regions to the temporoparietal/prefrontal cortices, respectively. As cervical dystonia is associated with abnormally increased cerebellar connectivity with the sensorimotor cortex (5), the improvement of motor symptoms following the decrease in cerebellar connectivity after BoNT injections may be expected. Such an improvement is not seen in sleep disruption (52), which remains after repeated treatment. These data argue that although cerebellar overactivity could contribute to circadian dysfunction in dystonia, the pathways affected by BoNT are functionally and anatomically distinct from those involved in sleep, which is perhaps not unexpected, given that the BoNT injections are given at the focal peripheral sites where dystonia is manifest rather than as a systemic administration typical of oral or other parenteral forms of drug administration. Such divergent pathways would explain the previous work described here, which suggests that motor dysfunction and sleep impairments occur in parallel in the cerebellar circuit (134). Alternatively, these “divergent pathways” may in fact represent differential outcomes of cerebellar hyperconnectivity versus hyperactivity, though further work is needed to fully explore and distinguish these possibilities.
Nevertheless, sleep and dystonia appear to be linked in such a way that may highlight the interaction of sleep and the cerebellum. There is evidence that patients with dystonia display sleep impairments, but a causative relationship between dystonia and sleep dysregulation remains unclear. Sleep latency and REM latency are indeed elevated in patients with isolated cervical dystonia, indicative of some form of circadian disruption (62). Interestingly though, while some studies show that dystonic muscle activity disappears during REM sleep (62), results from a recent patient survey suggest that dystonia symptoms may in fact persist even during sleep (134). Does this indicate that the motor symptoms of dystonia are directly related to sleep dysfunction? Certainly, evidence exists that muscle activity in dystonia decreases during sleep, increasing only in the moments prior to wake (135, 136); this is in line with the synaptic homeostasis hypothesis, which suggests that synaptic activity is renormalized during sleep (137). Therefore, while it is possible that muscle overactivity during wake is corrected during sleep, this mechanism may be aberrant in those with dystonia. A similar aberrant mechanism may exist at the level of the cerebellum. Normal sleep-dependent cerebello-thalamo-cortical communication and Purkinje cell activity is likely disrupted in patients with dystonia and may be particularly difficult to compensate for given the inability of patients to adequately manage sleep disruption. In this way, cerebellar dysfunction and sleep disruption in dystonia may each comprise two halves of a self-propelling cycle.
Discussion
There is substantial evidence in humans and animal models that convincingly implicates the cerebellum as a major node in the network disruptions underlying dystonia (5, 9, 10, 27). Furthermore, it is suggested that this disruption forms the basis for the comorbidities faced by dystonia patients beyond canonical motor symptoms, of which many physiological processes are involved, notably sleep (14, 15, 48, 51, 55, 61). While the pathogenesis for the involvement of the cerebellum in the development of dystonia remains an active area of investigation, Purkinje cells and cerebellar nuclei neurons are both definitively involved in the propagation of aberrant signals within and outside of the cerebellum—the cerebellar nuclei, in particular, have emerged as a common target for the modulation of motor symptoms (10, 109). Based on the reviewed evidence, it seems reasonable to propose that one or both of these cell types are involved in the complex relationship between the cerebellum and the major circadian centers of the brain, though the full mechanism by which the cerebellum contributes to circadian regulation remains elusive. Ultimately though, the relationship between sleep and the cerebellum in dystonia presents a unique opportunity to develop improved therapies that can help simultaneously treat both the motor and the nonmotor symptoms of the disorder, which as of yet has not been achieved with any single treatment modality.
It could be that targeted treatments like deep brain stimulation (DBS) could positively impact sleep disruptions, succeeding where other treatments (i.e., BoNT, drugs) have failed. BoNT therapy targets motor symptoms of dystonia by directly paralyzing affected muscles, administered at the site of motor dysfunction and hypothesized to function via retrograde transport to the CNS through motor axons (138). While effective, BoNT therapy is highly specific for alleviating motor dysfunction, due to its focal application. Although, while certain drugs like benzodiazepines or anticholinergics can be used to mitigate the motor symptoms of dystonia (139), they typically also have significant effects on sleep (140, 141). In contrast, one way that DBS is thought to function is by causing an “informational lesion” of synapses (142), which could be used to correct convergent sites of dysfunction, for example, at the cerebellar nuclei. This is a promising avenue, as DBS has been demonstrated to improve sleep (143, 144, 145, 146), a highly systemic process, and the technological capability to pair stimulation paradigms with sleep states is also proven (145) (though not in cases of isolated idiopathic dystonia). Understanding the sleep-cerebellum-dystonia relationship could also improve the benefits of non-invasive therapies, perhaps by incorporating a set pattern of rhythmicity during treatment administration, as has been achieved in cancer therapies (147, 148, 149).
Many open questions surround sleep, dystonia, and the cerebellum. For instance, while it is known that sleep disturbances exist across different motor disorders (13), it is likely that the sleep dysfunctions in each disease arise from unique causes, which could make a single universal treatment challenging. This would be especially true if the cellular and/or molecular mechanisms that are affected are different for each condition. However, if the circuit-level defects have some common loci–for example, the cerebellum–one could argue that therapeutics which target specific regions or circuits could have great benefits. Still, in the treatment of dystonia, such an approach could present a challenge, as “dystonia” encompasses a broad number of motor disorders with great heterogeneity across the different forms of the disease (2). Additionally, distinguishing between dystonia-dependent versus -independent sleep disturbances is difficult and will be an important obstacle to overcome both in the study of dystonia and the development of effective therapeutics. Even at the motor circuit level, distinguishing between the origins and impact of dystonia-related symptoms and the sleep problems (for example, are the sleep disturbances due to dystonic motor defects, or is their co-existence the indication of broader dysfunction?) is challenging. Moreover, stress and anxiety, which are known to have unique relationships with sleep and the circadian system (150), are notable non-motor symptoms in dystonia that are typically unaddressed by common and often effective treatments like BoNT therapy (46, 52). Another question to resolve emerged with the existence of mixed evidence regarding the prevalence of sleep disorders in dystonia; a study of 24 patients with both primary and secondary dystonia showed similar sleep organization and architecture relative to controls (151). It is possible that the use of highly specific mouse models could help address some of these concerns, and perhaps thereafter, specific diagnostic tests could be implemented in order to better define what therapies are suitable for each patient’s symptoms.
Nevertheless, it is becoming increasingly apparent that sleep disturbances are not only common among those affected by motor disorders (13, 46, 51, 52, 59, 60, 61, 62, 135, 136, 152), but that these disturbances are more than secondary symptoms. If we are to deeply and better understand network disorders like dystonia, we must approach future investigations into the mechanisms and potential therapies of the condition with a mindset that places the non-motor complications, such as sleep, in the same hierarchy as the visible motor complications that remain at the forefront of investigation. Thus, further research needs to be performed, in animal models and human patients, to better understand the complex role(s) of the cerebellum in the numerous motor and non-motor features of dystonia. In this regard, we propose that the cerebellum, and its connections, is precisely one such region that may help identify these critical common neural mechanisms.
Author Contributions
LS and RS wrote and edited the paper.
Funding
This work was supported by Baylor College of Medicine (BCM), Texas Children’s Hospital, The Hamill Foundation, and the National Institutes of Neurological Disorders and Stroke (NINDS) R01NS100874, R01NS119301, and R01NS127435 to RS. Research reported in this publication was supported by the Eunice Kennedy Shriver National Institute of Child Health and Human Development of the National Institutes of Health under Award Number P50HD103555 for use of the Cell and Tissue Pathogenesis Core (BCM IDDRC). Support was also provided by a Dystonia Medical Research Foundation (DMRF) grant to RS.
Author Disclaimer
The content is solely the responsibility of the authors and does not necessarily represent the official views of the National Institutes of Health.
Conflict of Interest
The authors declare that the research was conducted in the absence of any commercial or financial relationships that could be construed as a potential conflict of interest.
Acknowledgments
We thank Sarah Donofrio and Dr. Jason Gill for comments on earlier drafts of the manuscript.
Abbreviations
CB, cerebellum; DRN, dorsal raphe nucleus; LC, locus coeruleus; PBN, parabrachial nucleus; PPN, pedunculopontine nucleus; PC, Purkinje cell; SCN, suprachiasmatic nucleus.
References
1. Jinnah, HA, and Factor, SA. Diagnosis and Treatment of Dystonia. Neurol Clin (2015) 33:77–100. doi:10.1016/j.ncl.2014.09.002
2. Balint, B, Mencacci, NE, Valente, EM, Pisani, A, Rothwell, J, Jankovic, J, et al. Dystonia. Nat Rev Dis Primer (2018) 4:25. doi:10.1038/s41572-018-0023-6
3. Marsden, CD, Obeso, JA, Zarranz, JJ, and Lang, AE. The Anatomical Basis of Symptomatic Hemidystonia. Brain (1985) 108:463–83. doi:10.1093/brain/108.2.463
4. Wichmann, T, and DeLong, MR. Deep-Brain Stimulation for Basal Ganglia Disorders. Basal Ganglia (2011) 1:65–77. doi:10.1016/j.baga.2011.05.001
5. Corp, DT, Joutsa, J, Darby, RR, Delnooz, CCS, van de Warrenburg, BPC, Cooke, D, et al. Network Localization of Cervical Dystonia Based on Causal Brain Lesions. Brain (2019) 142:1660–74. doi:10.1093/brain/awz112
6. Hanekamp, S, and Simonyan, K. The Large-Scale Structural Connectome of Task-specific Focal Dystonia. Hum Brain Mapp (2020) 41:3253–65. doi:10.1002/hbm.25012
7. Hendrix, CM, and Vitek, JL. Toward a Network Model of Dystonia. Ann N Y Acad Sci (2012) 1265:46–55. doi:10.1111/j.1749-6632.2012.06692.x
8. Sadnicka, A, Hoffland, BS, Bhatia, KP, van de Warrenburg, BP, and Edwards, MJ. The Cerebellum in Dystonia - Help or Hindrance? Clin Neurophysiol (2012) 123:65–70. doi:10.1016/j.clinph.2011.04.027
9. Campbell, DB, North, JB, and Hess, EJ. Tottering Mouse Motor Dysfunction Is Abolished on the Purkinje Cell Degeneration (Pcd) Mutant Background. Exp Neurol (1999) 160:268–78. doi:10.1006/exnr.1999.7171
10. White, JJ, and Sillitoe, RV. Genetic Silencing of Olivocerebellar Synapses Causes Dystonia-like Behaviour in Mice. Nat Commun (2017) 8:14912. doi:10.1038/ncomms14912
11. Hess, EJ, and Jinnah, HA. Chapter 27-Mouse Models of Dystonia. In: MS LeDoux, editor. Movement Disorders. 2nd ed. Massachusetts, US: Academic Press (2015). p. 465–81. doi:10.1016/B978-0-12-405195-9.00027-5
12. Fuchs, T, Gavarini, S, Saunders-Pullman, R, Raymond, D, Ehrlich, ME, Bressman, SB, et al. Mutations in the THAP1 Gene Are Responsible for DYT6 Primary Torsion Dystonia. Nat Genet (2009) 41:286–8. doi:10.1038/ng.304
13. Song, B, and Zhu, J-C. A Narrative Review of Cerebellar Malfunctions and Sleep Disturbances. Front Neurosci (2021) 15:590619. doi:10.3389/fnins.2021.590619
14. Mendoza, J, Pévet, P, Felder-Schmittbuhl, M-P, Bailly, Y, and Challet, E. The Cerebellum Harbors a Circadian Oscillator Involved in Food Anticipation. J Neurosci (2010) 30:1894–904. doi:10.1523/JNEUROSCI.5855-09.2010
15. Xu, W, De Carvalho, F, Clarke, AK, and Jackson, A. Communication from the Cerebellum to the Neocortex during Sleep Spindles. Prog Neurobiol (2021) 199:101940. doi:10.1016/j.pneurobio.2020.101940
16. Napper, RM, and Harvey, RJ. Number of Parallel Fiber Synapses on an Individual Purkinje Cell in the Cerebellum of the Rat. J Comp Neurol (1988) 274:168–77. doi:10.1002/cne.902740204
17. Eccles, JC, Ito, M, and Szentágothai, J. The Parallel Fibers. In: The Cerebellum as a Neuronal Machine. Berlin, Germany: Springer (1967). p. 43–57. doi:10.1007/978-3-662-13147-3_4
18. Zang, Y, and De Schutter, E. Climbing Fibers Provide Graded Error Signals in Cerebellar Learning. Front Syst Neurosci (2019) 13:46. doi:10.3389/fnsys.2019.00046
19. Carta, I, Chen, CH, Schott, AL, Dorizan, S, and Khodakhah, K. Cerebellar Modulation of the Reward Circuitry and Social Behavior. Science (2019) 363:eaav0581. doi:10.1126/science.aav0581
20. Heffley, W, and Hull, C. Classical Conditioning Drives Learned Reward Prediction Signals in Climbing Fibers across the Lateral Cerebellum. eLife (2019) 8:e46764. doi:10.7554/eLife.46764
21. Heffley, W, Song, EY, Xu, Z, Taylor, BN, Hughes, MA, McKinney, A, et al. Coordinated Cerebellar Climbing Fiber Activity Signals Learned Sensorimotor Predictions. Nat Neurosci (2018) 21:1431–41. doi:10.1038/s41593-018-0228-8
22. Schilling, K, Oberdick, J, Rossi, F, and Baader, SL. Besides Purkinje Cells and Granule Neurons: an Appraisal of the Cell Biology of the Interneurons of the Cerebellar Cortex. Histochem Cel Biol (2008) 130:601–15. doi:10.1007/s00418-008-0483-y
23. Consalez, GG, Goldowitz, D, Casoni, F, and Hawkes, R. Origins, Development, and Compartmentation of the Granule Cells of the Cerebellum. Front Neural Circuits (2020) 14:611841. doi:10.3389/fncir.2020.611841
24. Purves, D, Augustine, GJ, and Fitzpatrick, D. Circuits within the Cerebellum. In: Neuroscience. 2nd ed. Sunderland, MA: Sinauer Associates (2001).
25. Floris, A, Diño, M, Jacobowitz, DM, and Mugnaini, E. The Unipolar brush Cells of the Rat Cerebellar Cortex and Cochlear Nucleus Are Calretinin-Positive: a Study by Light and Electron Microscopic Immunocytochemistry. Anat Embryol (1994) 189:495–520. doi:10.1007/BF00186824
26. Baumel, Y, Jacobson, G, and Cohen, D. Implications of Functional Anatomy on Information Processing in the Deep Cerebellar Nuclei. Front Cel Neurosci (2009) 3:14. doi:10.3389/neuro.03.014.2009
27. Ozelius, L, and Lubarr, N. DYT1 Early-Onset Isolated Dystonia. In: MP Adam, editor. GeneReviews®. Seattle, Washington: University of Washington (1993).
28. Naismith, TV, Dalal, S, and Hanson, PI. Interaction of torsinA with its Major Binding Partners Is Impaired by the Dystonia-Associated DeltaGAG Deletion. J Biol Chem (2009) 284:27866–74. doi:10.1074/jbc.M109.020164
29. Brown, RSH, Zhao, C, Chase, AR, Wang, J, and Schlieker, C. The Mechanism of Torsin ATPase Activation. Proc Natl Acad Sci U S A (2014) 111:E4822–31. doi:10.1073/pnas.1415271111
30. Li, J, Levin, DS, Kim, AJ, Pappas, SS, and Dauer, WT. TorsinA Restoration in a Mouse Model Identifies a Critical Therapeutic Window for DYT1 Dystonia. J Clin Invest (2021) 131:e139606. doi:10.1172/jci139606
31. Fremont, R, Tewari, A, Angueyra, C, and Khodakhah, K. A Role for Cerebellum in the Hereditary Dystonia DYT1. eLife (2017) 6:e22775. doi:10.7554/eLife.22775
32. van der Heijden, ME, Kizek, DJ, Perez, R, Ruff, EK, Ehrlich, ME, and Sillitoe, RV. Abnormal Cerebellar Function and Tremor in a Mouse Model for Non-manifesting Partially Penetrant Dystonia Type 6. J Physiol (2021) 599:2037–54. doi:10.1113/JP280978
33. Washburn, S, Fremont, R, Moreno-Escobar, MC, Angueyra, C, and Khodakhah, K. Acute Cerebellar Knockdown of Sgce Reproduces Salient Features of Myoclonus-Dystonia (DYT11) in Mice. eLife (2019) 8:e52101. doi:10.7554/eLife.52101
34. Fremont, R, Tewari, A, and Khodakhah, K. Aberrant Purkinje Cell Activity Is the Cause of Dystonia in a shRNA-Based Mouse Model of Rapid Onset Dystonia-Parkinsonism. Neurobiol Dis (2015) 82:200–12. doi:10.1016/j.nbd.2015.06.004
35. Zakirova, Z, Fanutza, T, Bonet, J, Readhead, B, Zhang, W, Yi, Z, et al. Mutations in THAP1/DYT6 Reveal that Diverse Dystonia Genes Disrupt Similar Neuronal Pathways and Functions. Plos Genet (2018) 14:e1007169. doi:10.1371/journal.pgen.1007169
36. Beauvais, G, Bode, NM, Watson, JL, Wen, H, Glenn, KA, Kawano, H, et al. Disruption of Protein Processing in the Endoplasmic Reticulum of DYT1 Knock-In Mice Implicates Novel Pathways in Dystonia Pathogenesis. J Neurosci (2016) 36:10245–56. doi:10.1523/JNEUROSCI.0669-16.2016
37. Hok, P, Hvizdosova, L, Otruba, P, Kaiserova, M, Trneckova, M, Tudos, Z, et al. Botulinum Toxin Injection Changes Resting State Cerebellar Connectivity in Cervical Dystonia. Sci Rep (2021) 11:8322. doi:10.1038/s41598-021-87088-z
38. Solstrand Dahlberg, L, Lungu, O, and Doyon, J. Cerebellar Contribution to Motor and Non-motor Functions in Parkinson’s Disease: A Meta-Analysis of fMRI Findings. Front Neurol (2020) 11:127. doi:10.3389/fneur.2020.00127
39. Isope, P, and Barbour, B. Properties of Unitary Granule cell-->Purkinje Cell Synapses in Adult Rat Cerebellar Slices. J Neurosci (2002) 22:9668–78. doi:10.1523/jneurosci.22-22-09668.2002
40. Ekerot, C-F, and Jörntell, H. Parallel Fibre Receptive fields of Purkinje Cells and Interneurons Are Climbing Fibre-specific. Eur J Neurosci (2001) 13:1303–10. doi:10.1046/j.0953-816x.2001.01499.x
41. Jörntell, H, and Ekerot, C-F. Reciprocal Bidirectional Plasticity of Parallel Fiber Receptive Fields in Cerebellar Purkinje Cells and Their Afferent Interneurons. Neuron (2002) 34:797–806. doi:10.1016/s0896-6273(02)00713-4
42. Dean, P, Porrill, J, Ekerot, C-F, and Jörntell, H. The Cerebellar Microcircuit as an Adaptive Filter: Experimental and Computational Evidence. Nat Rev Neurosci (2010) 11:30–43. doi:10.1038/nrn2756
43. Sokolov, AA, Miall, RC, and Ivry, RB. The Cerebellum: Adaptive Prediction for Movement and Cognition. Trends Cogn Sci (2017) 21:313–32. doi:10.1016/j.tics.2017.02.005
44. Calderon, DP, Fremont, R, Kraenzlin, F, and Khodakhah, K. The Neural Substrates of Rapid-Onset Dystonia-Parkinsonism. Nat Neurosci (2011) 14:357–65. doi:10.1038/nn.2753
45. Li, J, Liang, C-C, Pappas, SS, and Dauer, WT. TorsinB Overexpression Prevents Abnormal Twisting in DYT1 Dystonia Mouse Models. eLife (2020) 9:e54285. doi:10.7554/eLife.54285
46. Yang, J, Shao, N, Song, W, Wei, Q, Ou, R, Wu, Y, et al. Nonmotor Symptoms in Primary Adult-Onset Cervical Dystonia and Blepharospasm. Brain Behav (2016) 7:e00592. doi:10.1002/brb3.592
47. Duane, DD, and Bakken, EC. Additional Clinical Observations on Psychiatric Disorders in Adult-Onset Focal Dystonia: a Case Control Study. Mov Disord (2011) 26:1572. doi:10.1002/mds.23493
48. Bradnam, LV, Meiring, RM, Boyce, M, and McCambridge, A. Neurorehabilitation in Dystonia: a Holistic Perspective. J Neural Transm (2021) 128:549–58. doi:10.1007/s00702-020-02265-0
49. Ceylan, D, Erer, S, Zarifoğlu, M, Türkeş, N, and Özkaya, G. Evaluation of Anxiety and Depression Scales and Quality of LIFE in Cervical Dystonia Patients on Botulinum Toxin Therapy and Their Relatives. Neurol Sci (2019) 40:725–31. doi:10.1007/s10072-019-3719-9
50. Fabbrini, G, Berardelli, I, Moretti, G, Pasquini, M, Bloise, M, Colosimo, C, et al. Psychiatric Disorders in Adult-Onset Focal Dystonia: a Case-Control Study. Mov Disord (2010) 25:459–65. doi:10.1002/mds.22983
51. Eichenseer, SR, Stebbins, GT, and Comella, CL. Beyond a Motor Disorder: A Prospective Evaluation of Sleep Quality in Cervical Dystonia. Parkinsonism Relat Disord (2014) 20:405–8. doi:10.1016/j.parkreldis.2014.01.004
52. Costanzo, M, Belvisi, D, Berardelli, I, Maraone, A, Baione, V, Ferrazzano, G, et al. Effect of Botulinum Toxin on Non-motor Symptoms in Cervical Dystonia. Toxins (2021) 13:647. doi:10.3390/toxins13090647
53. Jackman, SL, Chen, CH, Offermann, HL, Drew, IR, Harrison, BM, Bowman, AM, et al. Cerebellar Purkinje Cell Activity Modulates Aggressive Behavior. eLife (2020) 9:e53229. doi:10.7554/eLife.53229
54. Baumann, O, and Mattingley, JB. Functional Topography of Primary Emotion Processing in the Human Cerebellum. NeuroImage (2012) 61:805–11. doi:10.1016/j.neuroimage.2012.03.044
55. Zhang, L-B, Zhang, J, Sun, MJ, Chen, H, Yan, J, Luo, FL, et al. Neuronal Activity in the Cerebellum during the Sleep-Wakefulness Transition in Mice. Neurosci Bull (2020) 36:919–31. doi:10.1007/s12264-020-00511-9
56. Van Overwalle, F, Baetens, K, Mariën, P, and Vandekerckhove, M. Social Cognition and the Cerebellum: a Meta-Analysis of over 350 fMRI Studies. NeuroImage (2014) 86:554–72. doi:10.1016/j.neuroimage.2013.09.033
57. Rowland, NC, Goldberg, JA, and Jaeger, D. Cortico-cerebellar Coherence and Causal Connectivity during Slow-Wave Activity. Neuroscience (2010) 166:698–711. doi:10.1016/j.neuroscience.2009.12.048
58. Canto, CB, Onuki, Y, Bruinsma, B, Werf, YD, and Zeeuw, CID. The Sleeping Cerebellum. Trends Neurosci (2017) 40:309–23. doi:10.1016/j.tins.2017.03.001
59. Smit, M, Kamphuis, ASJ, Bartels, AL, Han, V, Stewart, RE, Zijdewind, I, et al. Fatigue, Sleep Disturbances, and Their Influence on Quality of Life in Cervical Dystonia Patients. Mov Disord Clin Pract (2017) 4:517–23. doi:10.1002/mdc3.12459
60. Wagle Shukla, A, Wagle ShuklA, A, Heese, K, Jones, J, Rodriguez, RL, Malaty, IM, et al. High Rates of Fatigue and Sleep Disturbances in Dystonia. Int J Neurosci (2016) 126:928–35. doi:10.3109/00207454.2015.1085035
61. Hertenstein, E, Tang, NKY, Bernstein, CJ, Nissen, C, Underwood, MR, and Sandhu, HK. Sleep in Patients with Primary Dystonia: A Systematic Review on the State of Research and Perspectives. Sleep Med Rev (2016) 26:95–107. doi:10.1016/j.smrv.2015.04.004
62. Antelmi, E, Ferri, R, Provini, F, Scaglione, CML, Mignani, F, Rundo, F, et al. Modulation of the Muscle Activity during Sleep in Cervical Dystonia. Sleep (2017) 40:zsx088. doi:10.1093/sleep/zsx088
63. Hastings, MH, Maywood, ES, and Brancaccio, M. Generation of Circadian Rhythms in the Suprachiasmatic Nucleus. Nat Rev Neurosci (2018) 19:453–69. doi:10.1038/s41583-018-0026-z
64. Takahashi, JS. Transcriptional Architecture of the Mammalian Circadian Clock. Nat Rev Genet (2017) 18:164–79. doi:10.1038/nrg.2016.150
65. Mistlberger, RE. Neurobiology of Food Anticipatory Circadian Rhythms. Physiol Behav (2011) 104:535–45. doi:10.1016/j.physbeh.2011.04.015
66. Buhr, ED, Yoo, S-H, and Takahashi, JS. Temperature as a Universal Resetting Cue for Mammalian Circadian Oscillators. Science (2010) 330:379–85. doi:10.1126/science.1195262
67. Oster, H, Challet, E, Ott, V, Arvat, E, de Kloet, ER, Dijk, DJ, et al. The Functional and Clinical Significance of the 24-Hour Rhythm of Circulating Glucocorticoids. Endocr Rev (2017) 38:3–45. doi:10.1210/er.2015-1080
68. Mavroudis, PD, Scheff, JD, Calvano, SE, Lowry, SF, and Androulakis, IP. Entrainment of Peripheral Clock Genes by Cortisol. Physiol Genomics (2012) 44:607–21. doi:10.1152/physiolgenomics.00001.2012
69. Mohawk, JA, Green, CB, and Takahashi, JS. Central and Peripheral Circadian Clocks in Mammals. Annu Rev Neurosci (2012) 35:445–62. doi:10.1146/annurev-neuro-060909-153128
70. Pellman, BA, Kim, E, Reilly, M, Kashima, J, Motch, O, de la Iglesia, HO, et al. Time-Specific Fear Acts as a Non-photic Entraining Stimulus of Circadian Rhythms in Rats. Sci Rep (2015) 5:14916. doi:10.1038/srep14916
71. Ralph, MR, Foster, RG, Davis, FC, and Menaker, M. Transplanted Suprachiasmatic Nucleus Determines Circadian Period. Science (1990) 247:975–8. doi:10.1126/science.2305266
72. Boudreau, P, Dumont, GA, and Boivin, DB. Circadian Adaptation to Night Shift Work Influences Sleep, Performance, Mood and the Autonomic Modulation of the Heart. PLoS One (2013) 8:e70813. doi:10.1371/journal.pone.0070813
73. Violanti, JM, Charles, LE, Hartley, TA, Mnatsakanova, A, Andrew, ME, Fekedulegn, D, et al. Shift-work and Suicide Ideation Among Police Officers. Am J Ind Med (2008) 51:758–68. doi:10.1002/ajim.20629
74. Fu, L, and Kettner, NM. The Circadian Clock in Cancer Development and Therapy. Prog Mol Biol Transl Sci (2013) 119:221–82. doi:10.1016/B978-0-12-396971-2.00009-9
75. Sancar, A, Lindsey-Boltz, LA, Gaddameedhi, S, Selby, CP, Ye, R, Chiou, YY, et al. Circadian Clock, Cancer, and Chemotherapy. Biochemistry (2015) 54:110–23. doi:10.1021/bi5007354
76. Pimenta, AM, Kac, G, Souza, RRCE, Ferreira, LM, de, BA, and Silqueira, SM. Night-shift Work and Cardiovascular Risk Among Employees of a Public university. Revista da Associacao Med Brasileira (2012) 58:168–77. doi:10.1016/s0104-4230(12)70177-x
77. Brown, DL, Feskanich, D, Sanchez, BN, Rexrode, KM, Schernhammer, ES, and Lisabeth, LD. Rotating Night Shift Work and the Risk of Ischemic Stroke. Am J Epidemiol (2009) 169:1370–7. doi:10.1093/aje/kwp056
78. Reeves, BC, Karimy, JK, Kundishora, AJ, Mestre, H, Cerci, HM, Matouk, C, et al. Glymphatic System Impairment in Alzheimer’s Disease and Idiopathic Normal Pressure Hydrocephalus. Trends Mol Med (2020) 26:285–95. doi:10.1016/j.molmed.2019.11.008
79. Owen, JE, Zhu, Y, Fenik, P, Zhan, G, Bell, P, Liu, C, et al. Late-in-life Neurodegeneration after Chronic Sleep Loss in Young Adult Mice. Sleep (2021) 44:zsab057. doi:10.1093/sleep/zsab057
80. Spira, AP, Gamaldo, AA, An, Y, Wu, MN, Simonsick, EM, Bilgel, M, et al. Self-reported Sleep and β-amyloid Deposition in Community-Dwelling Older Adults. JAMA Neurol (2013) 70:1537–43. doi:10.1001/jamaneurol.2013.4258
81. Robertson, EM, Pascual-Leone, A, and Press, DZ. Awareness Modifies the Skill-Learning Benefits of Sleep. Curr Biol (2004) 14:208–12. doi:10.1016/j.cub.2004.01.027
82. Al-Sharman, A, and Siengsukon, CF. Sleep Enhances Learning of a Functional Motor Task in Young Adults. Phys Ther (2013) 93:1625–35. doi:10.2522/ptj.20120502
83. Walker, MP, Brakefield, T, Seidman, J, Morgan, A, Hobson, JA, and Stickgold, R. Sleep and the Time Course of Motor Skill Learning. Learn Mem (2003) 10:275–84. doi:10.1101/lm.58503
84. Zeeuw, DCI, and Canto, CB. Sleep Deprivation Directly Following Eyeblink-Conditioning Impairs Memory Consolidation. Neurobiol Learn Mem (2020) 170:107165. doi:10.1016/j.nlm.2020.107165
85. Cheng, LY, Che, T, Tomic, G, Slutzky, MW, and Paller, KA. Memory Reactivation during Sleep Improves Execution of a Challenging Motor Skill. J Neurosci (2021) 41:9608–16. doi:10.1523/JNEUROSCI.0265-21.2021
86. Siengsukon, CF, and Boyd, LA. Sleep Enhances Implicit Motor Skill Learning in Individuals Poststroke. Top Stroke Rehabil (2008) 15:1–12. doi:10.1310/tsr1501-1
87. Marchesi, GF, and Strata, P. Mossy and Climbing Fiber Activity during Phasic and Tonic Phenomena of Sleep. Pflugers Arch (1971) 323:219–40. doi:10.1007/BF00586385
88. Binda, F, Valente, P, Marte, A, Baldelli, P, and Benfenati, F. Increased Responsiveness at the Cerebellar Input Stage in the PRRT2 Knockout Model of Paroxysmal Kinesigenic Dyskinesia. Neurobiol Dis (2021) 152:105275. doi:10.1016/j.nbd.2021.105275
89. Cunchillos, JD, and De Andrés, I. Participation of the Cerebellum in the Regulation of the Sleep-Wakefulness Cycle. Results in Cerebellectomized Cats. Electroencephalogr Clin Neurophysiol (1982) 53:549–58. doi:10.1016/0013-4694(82)90067-0
90. De Andrés, I, Garzón, M, and Reinoso-Suárez, F. Functional Anatomy of Non-REM Sleep. Front Neurol (2011) 2:70. doi:10.3389/fneur.2011.00070
91. Acosta-Galvan, G, Yi, CX, van der Vliet, J, Jhamandas, JH, Panula, P, Angeles-Castellanos, M, et al. Interaction between Hypothalamic Dorsomedial Nucleus and the Suprachiasmatic Nucleus Determines Intensity of Food Anticipatory Behavior. Proc Natl Acad Sci U S A (2011) 108:5813–8. doi:10.1073/pnas.1015551108
92. Kornmann, B, Schaad, O, Bujard, H, Takahashi, JS, and Schibler, U. System-Driven and Oscillator-dependent Circadian Transcription in Mice with a Conditionally Active Liver Clock. Plos Biol (2007) 5:e34. doi:10.1371/journal.pbio.0050034
93. Bering, T, Hertz, H, and Rath, MF. Rhythmic Release of Corticosterone Induces Circadian Clock Gene Expression in the Cerebellum. Neuroendocrinology (2020) 110:604–15. doi:10.1159/000503720
94. Bering, T, Hertz, H, and Rath, MF. The Circadian Oscillator of the Cerebellum: Triiodothyronine Regulates Clock Gene Expression in Granule Cells In Vitro and in the Cerebellum of Neonatal Rats In Vivo. Front Physiol (2021) 12:706433. doi:10.3389/fphys.2021.706433
95. Kang, S, Jun, S, Baek, SJ, Park, H, Yamamoto, Y, and Tanaka-Yamamoto, K. Recent Advances in the Understanding of Specific Efferent Pathways Emerging from the Cerebellum. Front Neuroanat (2021) 15:759948. doi:10.3389/fnana.2021.759948
96. Carter, ME, Yizhar, O, Chikahisa, S, Nguyen, H, Adamantidis, A, Nishino, S, et al. Tuning Arousal with Optogenetic Modulation of Locus Coeruleus Neurons. Nat Neurosci (2010) 13:1526–33. doi:10.1038/nn.2682
97. Swift, KM, Gross, BA, Frazer, MA, Bauer, DS, Clark, KJD, Vazey, EM, et al. Abnormal Locus Coeruleus Sleep Activity Alters Sleep Signatures of Memory Consolidation and Impairs Place Cell Stability and Spatial Memory. Curr Biol (2018) 28:3599–609. doi:10.1016/j.cub.2018.09.054
98. Breton-Provencher, V, Drummond, GT, and Sur, M. Locus Coeruleus Norepinephrine in Learned Behavior: Anatomical Modularity and Spatiotemporal Integration in Targets. Front. Neural Circuits (2021) 15.
99. Hoffer, BJ, Siggins, GR, Oliver, AP, and Bloom, FE. Activation of the Pathway from Locus Coeruleus to Rat Cerebellar Purkinje Neurons: Pharmacological Evidence of Noradrenergic central Inhibition. J Pharmacol Exp Ther (1973) 184:553–69.
100. Moises, HC, Waterhouse, BD, and Woodward, DJ. Locus Coeruleus Stimulation Potentiates Purkinje Cell Responses to Afferent Input: the Climbing Fiber System. Brain Res (1981) 222:43–64. doi:10.1016/0006-8993(81)90939-2
101. Schwarz, LA, Miyamichi, K, Gao, XJ, Beier, KT, Weissbourd, B, DeLoach, KE, et al. Viral-genetic Tracing of the Input-Output Organization of a central Noradrenaline Circuit. Nature (2015) 524:88–92. doi:10.1038/nature14600
102. Palmiter, RD. The Parabrachial Nucleus: CGRP Neurons Function as a General Alarm. Trends Neurosci (2018) 41:280–93. doi:10.1016/j.tins.2018.03.007
103. Anaclet, C, Lin, JS, Vetrivelan, R, Krenzer, M, Vong, L, Fuller, PM, et al. Identification and Characterization of a Sleep-Active Cell Group in the Rostral Medullary Brainstem. J Neurosci (2012) 32:17970–6. doi:10.1523/JNEUROSCI.0620-12.2012
104. Hayashi, Y, Kashiwagi, M, Yasuda, K, Ando, R, Kanuka, M, Sakai, K, et al. Cells of a Common Developmental Origin Regulate REM/non-REM Sleep and Wakefulness in Mice. Science (2015) 350:957–61. doi:10.1126/science.aad1023
105. Hashimoto, M, Yamanaka, A, Kato, S, Tanifuji, M, Kobayashi, K, and Yaginuma, H. Anatomical Evidence for a Direct Projection from Purkinje Cells in the Mouse Cerebellar Vermis to Medial Parabrachial Nucleus. Front Neural Circuits (2018) 12:6. doi:10.3389/fncir.2018.00006
106. Cui, S-Y, Li, SJ, Cui, XY, Zhang, XQ, Yu, B, Huang, YL, et al. Ca2+ in the Dorsal Raphe Nucleus Promotes Wakefulness via Endogenous Sleep-Wake Regulating Pathway in the Rats. Mol Brain (2016) 9:71. doi:10.1186/s13041-016-0252-0
107. Monti, JM. The Role of Dorsal Raphe Nucleus Serotonergic and Non-serotonergic Neurons, and of Their Receptors, in Regulating Waking and Rapid Eye Movement (REM) Sleep. Sleep Med Rev (2010) 14:319–27. doi:10.1016/j.smrv.2009.10.003
108. Monti, JM. Serotonin Control of Sleep-Wake Behavior. Sleep Med Rev (2011) 15:269–81. doi:10.1016/j.smrv.2010.11.003
109. Kim, JE, Chae, S, Kim, S, Jung, YJ, Kang, MG, Heo, WD, et al. Cerebellar 5HT-2A Receptor Mediates Stress-Induced Onset of Dystonia. Sci Adv (2021) 7:eabb5735. doi:10.1126/sciadv.abb5735
110. Krout, KE, Kawano, J, Mettenleiter, TC, and Loewy, AD. CNS Inputs to the Suprachiasmatic Nucleus of the Rat. Neuroscience (2002) 110:73–92. doi:10.1016/s0306-4522(01)00551-6
111. Romigi, A, Peppe, A, Pierantozzi, M, Izzi, F, Brusa, L, Galati, S, et al. Pedunculopontine Nucleus Stimulation Influences REM Sleep in Parkinson’s Disease. Eur J Neurol (2008) 15:e64–5. doi:10.1111/j.1468-1331.2008.02167.x
112. Rye, DB. Contributions of the Pedunculopontine Region to normal and Altered REM Sleep. Sleep (1997) 20:757–88. doi:10.1093/sleep/20.9.757
113. Urbano, FJ, D'Onofrio, SM, Luster, BR, Beck, PB, Hyde, JR, Bisagno, V, et al. Pedunculopontine Nucleus Gamma Band Activity-Preconscious Awareness, Waking, and REM Sleep. Front Neurol (2014) 5:210. doi:10.3389/fneur.2014.00210
114. French, IT, and Muthusamy, KAA. A Review of the Pedunculopontine Nucleus in Parkinson's Disease. Front Aging Neurosci (2018) 10:99. doi:10.3389/fnagi.2018.00099
115. Mente, K, Edwards, NA, Urbano, D, Ray-Chaudhury, A, Iacono, D, Alho, ATDL, et al. Pedunculopontine Nucleus Cholinergic Deficiency in Cervical Dystonia. Mov Disord (2018) 33:827–34. doi:10.1002/mds.27358
116. Van Dort, CJ, Zachs, DP, Kenny, JD, Zheng, S, Goldblum, RR, Gelwan, NA, et al. Optogenetic Activation of Cholinergic Neurons in the PPT or LDT Induces REM Sleep. Proc Natl Acad Sci U S A (2015) 112:584–9. doi:10.1073/pnas.1423136112
117. Eban-Rothschild, A, Rothschild, G, Giardino, WJ, Jones, JR, and de Lecea, L. VTA Dopaminergic Neurons Regulate Ethologically Relevant Sleep–Wake Behaviors. Nat Neurosci (2016) 19:1356–66. doi:10.1038/nn.4377
118. Cutando, L, Puighermanal, E, Castell, L, Tarot, P, Belle, M, Bertaso, F, et al. Cerebellar Dopamine D2 Receptors Regulate Social Behaviors. Nat Neurosci (2022) 25:900–11. doi:10.1038/s41593-022-01092-8
119. Neychev, VK, Gross, R, Lehéricy, S, Hess, EJ, and Jinnah, HA. The Functional Neuroanatomy of Dystonia. Neurobiol Dis (2011) 42:185–201. doi:10.1016/j.nbd.2011.01.026
120. Dietrichs, E, and Haines, DE. Interconnections between Hypothalamus and Cerebellum. Anat Embryol (1989) 179:207–20. doi:10.1007/BF00326585
121. Morin, LP. Neuroanatomy of the Extended Circadian Rhythm System. Exp Neurol (2013) 243:4–20. doi:10.1016/j.expneurol.2012.06.026
122. Mano, N. Changes of Simple and Complex Spike Activity of Cerebellar Purkinje Cells with Sleep and Waking. Science (1970) 170:1325–7. doi:10.1126/science.170.3964.1325
123. McCarley, RW, and Hobson, JA. Simple Spike Firing Patterns of Cat Cerebellar Purkinje Cells in Sleep and Waking. Electroencephalogr Clin Neurophysiol (1972) 33:471–83. doi:10.1016/0013-4694(72)90211-8
124. Herzfeld, DJ, Kojima, Y, Soetedjo, R, and Shadmehr, R. Encoding of Action by the Purkinje Cells of the Cerebellum. Nature (2015) 526:439–42. doi:10.1038/nature15693
125. Lydic, R. The Motor Atonia of REM Sleep: a Critical Topics Forum. Introduction. Sleep (2008) 31:1471–2. doi:10.1093/sleep/31.11.1471
126. Dang-Vu, TT, Schabus, M, Desseilles, M, Albouy, G, Boly, M, Darsaud, A, et al. Spontaneous Neural Activity during Human Slow Wave Sleep. Proc Natl Acad Sci U S A (2008) 105:15160–5. doi:10.1073/pnas.0801819105
127. Torres-Herraez, A, Watson, T, and Rondi-Reig, L. Delta Oscillations Coordinate Intra-cerebellar and Cerebello-Hippocampal Network Dynamics during Sleep. J Neurosci (2022) 42:2268–81. doi:10.1523/JNEUROSCI.1479-21.2021
128. Pisotta, I, and Molinari, M. Cerebellar Contribution to Feedforward Control of Locomotion. Front Hum Neurosci (2014) 8:475. doi:10.3389/fnhum.2014.00475
129. Blumberg, MS, Marques, HG, and Iida, F. Twitching in Sensorimotor Development from Sleeping Rats to Robots. Curr Biol (2013) 23:R532–7. doi:10.1016/j.cub.2013.04.075
130. Sokoloff, G, Uitermarkt, BD, and Blumberg, MSREM. REM Sleep Twitches Rouse Nascent Cerebellar Circuits: Implications for Sensorimotor Development. Dev Neurobiol (2015) 75:1140–53. doi:10.1002/dneu.22177
131. Heijdenvan der, ME, and Sillitoe, RV. Motor Control: Internalizing Your Place in the World. Curr Biol (2021) 31:R1576–R1578. doi:10.1016/j.cub.2021.10.056Brown
132. Sokoloff, G, Plumeau, AM, Mukherjee, D, and Blumberg, MS. Twitch-related and Rhythmic Activation of the Developing Cerebellar Cortex. J Neurophysiol (2015) 114:1746–56. doi:10.1152/jn.00284.2015
133. Dooley, JC, Sokoloff, G, and Blumberg, MS. Movements during Sleep Reveal the Developmental Emergence of a Cerebellar-dependent Internal Model in Motor Thalamus. Curr Biol (2021) 31:5501–11. doi:10.1016/j.cub.2021.10.014
134. Smith, B. Lived Experience with Dystonia (2021). Available from: https://dystoniasurveys.files.wordpress.com/2021/08/lived-experience-dystonia-patient-surveys-2020-2021.pdf.
135. Silvestri, R, De Domenico, P, Di Rosa, AE, Bramanti, P, Serra, S, and Di PeRRi, R. The Effect of Nocturnal Physiological Sleep on Various Movement Disorders. Mov Disord (1990) 5:8–14. doi:10.1002/mds.870050104
136. Sforza, E, Montagna, P, Defazio, G, and Lugaresi, E. Sleep and Cranial Dystonia. Electroencephalogr Clin Neurophysiol (1991) 79:166–9. doi:10.1016/0013-4694(91)90135-q
137. Tononi, G, and Cirelli, C. Sleep and the Price of Plasticity: From Synaptic and Cellular Homeostasis to Memory Consolidation and Integration. Neuron (2014) 81:12–34. doi:10.1016/j.neuron.2013.12.025
138. Pirazzini, M, Rossetto, O, Eleopra, R, and Montecucco, C. Botulinum Neurotoxins: Biology, Pharmacology, and Toxicology. Pharmacol Rev (2017) 69:200–35. doi:10.1124/pr.116.012658
139. Termsarasab, P, Thammongkolchai, T, and Frucht, SJ. Medical Treatment of Dystonia. J Clin Mov Disord (2016) 3:19. doi:10.1186/s40734-016-0047-6
140. Kumar, S, Hasan, SS, Wong, PS, Chong, DWK, and Kairuz, T. Anticholinergic Burden, Sleep Quality and Health Outcomes in Malaysian Aged Care Home Residents. Pharmacy (2019) 7:143. doi:10.3390/pharmacy7040143
141. de Mendonça, FMR, de Mendonca, GPRR, Souza, LC, Galvao, LP, Paiva, HS, de Azevedo Marques Perico, C, et al. Benzodiazepines and Sleep Architecture: a Systematic Review. CNS Neurol Disord Drug Targets (2021) 22:172–9. doi:10.2174/1871527320666210618103344
142. Lozano, AM, and Eltahawy, H. How Does DBS Work? Suppl Clin Neurophysiol (2004) 57:733–6. doi:10.1016/s1567-424x(09)70414-3
143. Amara, AW, Watts, RL, and Walker, HC. The Effects of Deep Brain Stimulation on Sleep in Parkinson’s Disease. Ther Adv Neurol Disord (2011) 4:15–24. doi:10.1177/1756285610392446
144. Bjerknes, S, Skogseid, IM, Hauge, TJ, Dietrichs, E, and Toft, M. Subthalamic Deep Brain Stimulation Improves Sleep and Excessive Sweating in Parkinson’s Disease. NPJ Parkinsons Dis (2020) 6:29–7. doi:10.1038/s41531-020-00131-0
145. Gilron, R, Little, S, Wilt, R, Perrone, R, Anso, J, and Starr, PA. Sleep-Aware Adaptive Deep Brain Stimulation Control: Chronic Use at Home with Dual Independent Linear Discriminate Detectors. Front Neurosci (2021) 15:732499. doi:10.3389/fnins.2021.732499
146. Zuzuárregui, JRP, and Ostrem, JL. The Impact of Deep Brain Stimulation on Sleep in Parkinson’s Disease: An Update. J Parkinsons Dis (2020) 10:393–404. doi:10.3233/JPD-191862
147. Kuo, TT, and Ladurner, AG. Exploiting the Circadian Clock for Improved Cancer Therapy: Perspective from a Cell Biologist. Front Genet (2019) 10:1210. doi:10.3389/fgene.2019.01210
148. Lee, Y. Roles of Circadian Clocks in Cancer Pathogenesis and Treatment. Exp Mol Med (2021) 53:1529–38. doi:10.1038/s12276-021-00681-0
149. Lévi, F, Okyar, A, Dulong, S, Innominato, PF, and Clairambault, J. Circadian Timing in Cancer Treatments. Annu Rev Pharmacol Toxicol (2010) 50:377–421. doi:10.1146/annurev.pharmtox.48.113006.094626
150. Koch, CE, Leinweber, B, Drengberg, BC, Blaum, C, and Oster, H. Interaction between Circadian Rhythms and Stress. Neurobiol Stress (2016) 6:57–67. doi:10.1016/j.ynstr.2016.09.001
151. Fish, DR, Allen, PJ, Sawyers, D, and Marsden, CD. Sleep Spindles in Torsion Dystonia. Arch Neurol (1990) 47:216–8. doi:10.1001/archneur.1990.00530020124024
Keywords: dystonia, sleep, circadian rhythms, Purkinje cells, cerebellar nuclei
Citation: Salazar Leon LE and Sillitoe RV (2022) Potential Interactions Between Cerebellar Dysfunction and Sleep Disturbances in Dystonia. Dystonia 1:10691. doi: 10.3389/dyst.2022.10691
Received: 06 June 2022; Accepted: 20 September 2022;
Published: 04 October 2022.
Edited by:
Aasef Shaikh, Case Western Reserve University, United StatesCopyright © 2022 Salazar Leon and Sillitoe. This is an open-access article distributed under the terms of the Creative Commons Attribution License (CC BY). The use, distribution or reproduction in other forums is permitted, provided the original author(s) and the copyright owner(s) are credited and that the original publication in this journal is cited, in accordance with accepted academic practice. No use, distribution or reproduction is permitted which does not comply with these terms.
*Correspondence: Roy V. Sillitoe, c2lsbGl0b2VAYmNtLmVkdQ==