- Department of Neurosciences, Case Western Reserve University, Cleveland, OH, United States
Advances in sequencing technologies have identified novel genes associated with inherited forms of dystonia, providing valuable insights into its genetic basis and revealing diverse genetic pathways and mechanisms involved in its pathophysiology. Since identifying genetic variation in the transcription factor coding THAP1 gene linked to isolated dystonia, numerous investigations have employed transcriptomic studies in DYT-THAP1 models to uncover pathogenic molecular mechanisms underlying dystonia. This review examines key findings from transcriptomic studies conducted on in vivo and in vitro DYT-THAP1 models, which demonstrate that the THAP1-regulated transcriptome is diverse and cell-specific, yet it is bound and co-regulated by a common set of proteins. Prominent among its functions, THAP1 and its co-regulatory network target molecular pathways critical for generating myelinating oligodendrocytes that ensheath axons and generate white matter in the central nervous system. Several lines of investigation have demonstrated the importance of myelination and oligodendrogenesis in motor function during development and in adults, emphasizing the non-cell autonomous contributions of glial cells to neural circuits involved in motor function. Further research on the role of myelin abnormalities in motor deficits in DYT6 models will enhance our understanding of axon-glia interactions in dystonia pathophysiology and provide potential therapeutic interventions targeting these pathways.
Introduction
Dystonia manifests as prolonged involuntary twisting movements that can occur in isolation or in combination with other neurological symptoms [1, 2]. In 2009, Fuchs et al. identified that loss-of-function mutations in THAP1, which encodes a transcription factor with a zinc finger DNA-binding domain, cause DYT6 dystonia [3]. Since this discovery, numerous investigations have focused on transcriptomic studies of DYT-THAP1 (also known as DYT6) models to uncover pathogenic molecular mechanisms underlying dystonia. Here, we comprehensively examine prior investigations aiming to identify the THAP1 transcriptional network in neural and non-neural lineage. Additionally, we discuss the critical role of THAP1 and its network members in regulating neuron-glia interactions and their significance in regulating motor function.
Studies of the THAP1 regulated transcriptome
THAP1, similar to most of the dystonia genes, is ubiquitously expressed in both neuronal and non-neuronal cells and within multiple regions of the CNS [4]. Hence the expression pattern makes it challenging to gauge its role in motor function. THAP1 was first discovered in a two-hybrid screen as an interactor of prostate-apoptosis-response-4 (Par-4) protein [5]. Gene expression studies from overexpression and knockdown of THAP1 in HUVEC cells demonstrated that THAP1 regulates cell cycle and apoptotic pathways by modulating pRb/E2F target genes [6]. Several studies have since tried to identify and elucidate a core function of the THAP1 in cells of neural origin. We have identified 10 unbiased transcriptomic studies in DYT6 models that are listed in Table 1. These studies include investigations of: a) brain tissue samples from motor related regions (cortex, striatum and cerebellum) derived from mouse models with THAP1 deletion or those carrying DYT6 pathogenic mutations from adult [8], juvenile [7] and neonatal animals [9]; b) neuronal cultures that include primary striatal cultures, induced pluripotent stem cell (iPSC) derived cortical neuron precursor, dopaminergic and medium spiny neurons [9, 12, 13, 17]; c) non-neuronal cells of neural lineage that include oligodendrocytes [14], neuroblastoma cell line (SH-SY5Y) [13, 18] and iPSC derived neural stem cells [11]; d) non-neural cell types including HUVEC [6], mouse embryonic stem cells (mESC) [10] and mouse embryonic fibroblast [15]. Additionally, THAP1-bound genomic regions have been defined using ChIP-seq in K562, mESC and SH-SY5Y cells [10, 13, 16].
In our recent study [19], we compared THAP1-regulated transcriptome datasets commonly from all motor related regions (cortex, striatum, and cerebellum) [7], and oligodendrocyte cultures [14], which are direct targets of THAP1 (ChIP-seq data from ENCODE) [16] to identify core genes regulated by THAP1 in vivo and in vitro from neuronal and non-neuronal cells. Only five genes (Ech1, Cuedc2, Dpagt1, Prepl, and Cln3) were commonly identified across these datasets [19]. These observations and comparisons of differentially expressed genes (DEGs) from studies listed in Table 1 argue for a largely cell-specific program and that the cellular context in which the transcriptomic study was conducted strongly influences the final list of THAP1-regulated genes [19]. Yet another surprising observation is that majority of the THAP1-bound genes are not differentially expressed from THAP1 loss-of-function. For instance, Aguilo et al. reported only 10% overlap between genes in mESC that are THAP1-bound (ChIP-seq) and differentially expressed (RNA-seq) upon Thap1 deletion [10] while Cheng et al. observed a mere 7.5% overlap of THAP1-bound and DEGs from mutant THAP1 in SH-SY5Y cells [13]. These findings suggest that THAP1 transcriptome may additionally depend on its co-regulators.
Co-regulators of the THAP1 transcription factor pathway
A core feature of transcription factors is that they function in complexes. What proteins function as co-regulators of THAP1 transcriptional activity? Unlike the diverse molecular pathways regulated by THAP1 in various studies, a specific set of co-regulators appears to be associated with THAP1. Notably, THAP1-bound promoters in both mouse and human genomes are co-bound with YY1 (YIN-YANG-1), a transcription factor belonging to the GLI-Kruppel class of zinc finger proteins [20]. Through in silico analyses of THAP1-bound genes (ENCODE), we first identified that >90% of the THAP1 bound promoters are co-bound by YY1 [7, 19]. Furthermore, THAP1 affects the genome occupancy of YY1 [7]. Loss of THAP1 protein ablates the binding of YY1 at the promoter of shared loci [7]. Multiple independent studies using diverse models have since confirmed THAP1 and YY1 association. Domingo et al. engineered an allelic series of eight disease mutations in a common iPSC background and differentiated these lines into near-isogenic neural stem cells [11]. In this study, they observed that DEGs from multiple allelic series were enriched for YY1 binding motifs [11]. Aguilo et al. performed THAP1 ChIP-seq in mESC, which identified significant enrichment of YY1 in THAP1-bound genomic regions [10]. Additionally, Baumann et al. conducted transcriptome analysis on cortical neuronal precursors derived from iPSC generated from individuals with manifesting and non-manifesting THAP1 mutation carriers. Their study revealed that the YY1 binding motif was the most enriched in DEGs from carriers with manifesting symptoms [12]. Thus, while the THAP1 transcriptome appears to be cell specific, they are co-bound with YY1 across multiple species and cellular contexts. Since the first description of YY1 as a binding partner of THAP1 [7], numerous studies have reported loss of function YY1 mutations in childhood onset isolated dystonia [21–24]. These reports from human patients suggest the importance of THAP1-YY1 association in dystonia pathophysiology.
Multiple independent studies, utilizing in silico analyses and functional testing have identified HCFC1 and SP1 as other putative co-regulators of THAP1 transcriptome [7, 13, 15, 19, 25]. HCFC1 is another transcription factor that co-localizes with majority of THAP1 and YY1 bound genes [7, 10, 25], suggesting these three transcription factors could exist as a co-regulatory module. Loss of THAP1 regulates the occupancy of HCFC-1, like YY1 [25]. Functional evidence of co-regulation by THAP1, YY1, and HCFC1 was provided by Shinoda et al., who discovered their direct binding to the SHLD1 promoter and their cooperative action in maintaining low basal expression of SHLD1, crucial for DNA double-strand break repair [15]. In an interesting study, Cheng et al. identified SP1, a zinc finger transcription factor as a key downstream regulator of THAP1 differentially expressed in SH-SY5Y cell lines [13]. Transcriptomic analyses revealed that SP1 is a target of THAP1 and largely responsible for its DEGs, thus representing an important critical downstream member of the THAP1 driven transcriptional regulatory network [13]. We are not including further description on several other singularly predicted THAP1 co-regulatory proteins from investigations listed in Table 1 or those defined from proteomic studies [26] as they are beyond the scope of this review.
Beyond these co-regulators, THAP1 genomic association is enriched in regions of active chromatin. Aguilo et al. conducted ChIP-seq for THAP1 in mESC and identified that THAP1-bound genomic regions is enriched for epigenetic signatures of active enhancers and promoters—which includes H3K4me1, H3K27ac and H3K4me3 [10]. Furthermore, these active state epigenetic marks are enriched with signature motifs of THAP1 co-regulatory factors. Cheng et al. observed that genomic regions showing THAP1-dependent changes in H3K4me3 and H3K27ac are enriched for SP1 binding motifs [13]. These findings provide valuable insights into the mechanisms governing gene expression and epigenetic regulation by THAP1.
Role of THAP1 co-regulators in disease mechanism
Pathogenic mutations in THAP1 have been reported to exhibit a penetrance of approximately 50%. However, the underlying factors responsible for incomplete penetrance remain largely elusive. In a recent study Dulovic-Mahlow et al., provided a clear evidence that the expression of THAP1 is not likely the source of the reduced penetrance [27]. The investigators in this study analyzed for changes in THAP1 and TOR1A expression in a multigenerational family with reduced penetrance (<10%) despite a loss-of-function nonsense THAP1 mutation (p. Arg29X). Affected and unaffected carriers similar expression for THAP1 and TOR1A [27]. Consistent with this patient data, we and others have observed and reported that THAP1 auto-regulates its expression. Thus, changes in the level of THAP1 is unlikely to explain the penetrance [28].
Could mechanisms related to THAP1’s transcriptional activity provide an explanation for reduced penetrance? In a recent study conducted by Baumann et al., differential gene expression analysis was employed on cortical precursor neurons derived from affected or manifesting carriers (MMCs) and unaffected or non-manifesting carriers (NMCs) in an attempt to identify a gene signature correlating with symptoms [12]. They identified DEGs and pathways exclusively upregulated in MMCs compared to NMCs. Neuronal signaling pathways, including dopamine transmitter and eIF2α signaling, as well as cortical network formation, were found to be upregulated in MMCs, while extracellular matrix organization and DNA methylation were upregulated in NMCs. Although the exact DEGs may be influenced by cell type and neuronal culture conditions, the underlying signature of transcription factors regulating these genes provides additional clues regarding the mechanism underlying MMC-specific pathways. The DEGs in MMCs were observed to be enriched for the binding signatures of YY1 (along with ARID2, MIER1, and ZBTB11), while NMC DEGs were associated with SIX2 [12]. These results suggest that the involvement of YY1 in the regulation of THAP1 dependent DEGs in the MMC. Additionally, THAP1 downstream target and co-regulator SP1 has also been reported to show increased expression in THAP1 patients’ iPSC-derived mDA neurons, while it is decreased in THAP1 patients’ frontal cortex [13]. As discussed in the prior section, THAP1 DEGs are enriched in active epigenetic modifications and enrichment. An active role of epigenetic status in penetrance, while yet to be demonstrated, is likely.
More than 70% of the reported THAP1 mutations consist of missense mutations, with a smaller proportion being indels or mutations causing early truncation [29–31]. The majority of missense mutations affect the N-terminal THAP domain and have been described in other review articles [31, 32]. However, up to this point, DYT6 mutations have been reported only in seven of the 13 DNA binding residues comprising of eight invariant ones (C5, C10, C54, H57, P26, W36, F58, and P78) and an additional five residues (K24, R29, R42, F45, and T48) (as depicted in Figure 1) [33–35]. Furthermore, disease mutations have been identified in the C-terminal domain, which affects the protein-interacting leucine zipper and coiled-coil domains, thus influencing hetero- and homo-dimerization and the overall protein structure [30, 36]. These findings suggest that loss-of-function DYT6 mutations in THAP1 also involve mechanisms not disrupting its DNA binding. In our recent studies, we reported that the F81L DYT6 mutation [3], which occurs in the N terminus of the protein but not the DNA binding residue, impairs THAP1’s transcriptional activity without affecting its genomic binding [19]. Instead, it disrupts the interactions between THAP1 and YY1 and results in decreased histone acetylation (H3K9ac) at THAP1 regulated loci [19]. These findings suggest an important regulatory role for YY1 in the DYT6 disease mechanism and provide insights into how co-regulatory proteins influence THAP1’s transcriptional activity.
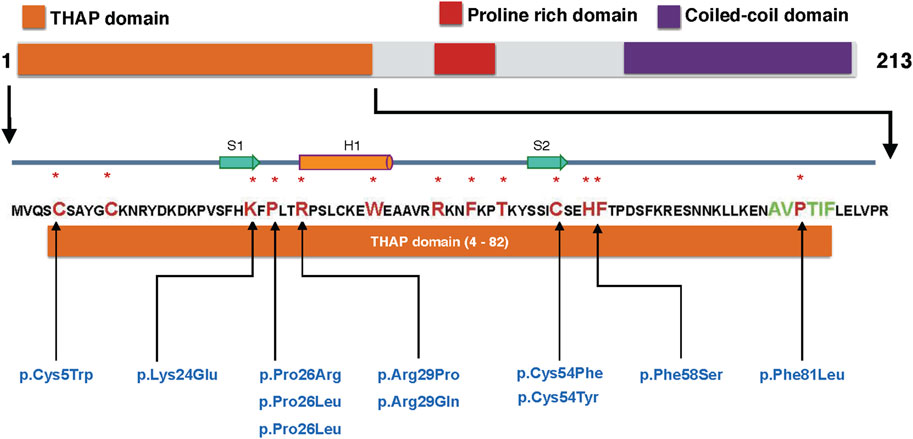
FIGURE 1. DYT6 mutations in DNA binding residues of THAP1: The schematic of the THAP1 protein illustrates its major domains: the THAP domain (responsible for DNA binding, depicted in orange), the proline-rich region (highlighted in red), and the coiled-coil domain (shown in purple). A magnified view of the N-terminal THAP domain (residues 4–82) is provided, with eight invariant residues (C5, C10, C54, H57, P26, W36, F58, and P78) and five additional residues (K24, R29, R42, F45 and T48) [33–35] involved in DNA binding indicated in larger font red and marked with a asterix. Additionally, the AVPTIF motif, highlighted in green, is presented. Missense mutations found in the DNA binding residues of THAP1, as identified from the MDSGene database (https://www.mdsgene.org), are listed in blue.
Regulation of axon-glia interactions by members of the THAP1 transcription factor pathway
In a recent study, Diaw et al. identified the most significant THAP1 dependent DEGs from existing studies [37]. They observed a rather diverse set of pathways, including myelination, nervous system development, cytoskeleton, neuron projection, dopamine signaling, and axonal guidance, among others [37]. Multiple other studies have also reported cell cycle, extra cellular matrix and cell adhesion among other THAP1-regulated pathways (references in Table 1), [6, 9, 12, 14, 15]. Neuron specific THAP1 pathways of note include GABAA receptor alpha2 in iPSC derived medium spiny neurons [17], eIF2α (Eukaryotic Initiation Factor 2 alpha) in striatal neurons [9], synaptic transmission commonly in rat striatal neurons and iPSC derived dopaminergic neurons [13]. Therefore, a wide range of biological pathways have been identified in the THAP1-regulated transcriptome across various studies (listed in Table 1). Of note among these pathways is myelination, a prominent process governing axon-glia interactions that is a target of THAP1, YY1 and SP1 [7, 38, 39].
Myelination pathway is commonly dysregulated in multiple transcriptomic datasets using both iPSC and mouse DYT6 models [7, 8, 11, 37]. Furthermore, myelination actively contributes to circuit plasticity, supporting motor learning [40–42]. Myelination is a postnatal process in which oligodendrocyte cells wrap axons with a myelin sheath to support rapid neurotransmission [43]. In the CNS, myelination is facilitated exclusively by the generation of mature myelinating oligodendrocytes, which are differentiated from oligodendrocyte progenitor cells (OPCs). The peak of myelination during development coincides with the peak of synaptogenesis, occurring mostly within the first 2 years in humans and the first 3 weeks in rodents [44–46].
Our prior work demonstrates that CNS conditional THAP1 null mice exhibit severe hypomyelination accompanied by juvenile-onset motor dysfunction persisting into adulthood [7]. Transmission electron microscopy (TEM) analysis of myelin ultrastructure in THAP1-ablated mice revealed a significant (>5-fold) reduction in the percentage of myelinated axons in white matter (WM) tracts in the juvenile CNS, along with a decrease (∼25%) in myelin thickness [7]. Similarly, the DYT6 mutant THAP1F81L, which represents a hypomorphic form of THAP1, also leads to CNS hypomyelination, as observed through TEM analysis [19]. Interestingly, myelination and oligodendrogenesis are also regulated by YY1. He et al. identified that conditional Yy1 ablation in vivo results in defective myelination in the CNS based on ultrastructure studies [38]. Both THAP1 and YY1 regulate CNS myelination through cell-autonomous role in the generation of myelinating oligodendrocyte (OL), without disrupting the fate of the parent OPC [7, 14, 19, 38]. Another THAP1 co-regulatory protein SP1, also has a critical role in myelination from its actions within the OL lineage. SP1 promotes OPC differentiation by aiding their exit from cell cycle and activating the transcription of the myelin basic protein [13, 39, 47–50]. In these studies, SP1 was demonstrated to directly bind and activate the Mbp loci [39, 48–50].
In addition to myelination abnormalities observed in animal models, changes in WM microstructure have been reported in patients with twelve different forms of inherited dystonia—either in isolation or combination with other neurological symptoms [4]. Eight of the mutated genes have an established role in myelination (THAP1, SLC2A1/GLUT1, BAP31, FA2H, SLC16A2/MCT8, YY1, POLR3 and TUBB4A) [4]. Several additional studies have reported microstructural WM changes in idiopathic dystonia’s [51]. More details regarding the genetic links between WM abnormalities and myelination in various forms of dystonia have been discussed elsewhere [4].
Role of myelination and oligodendrogenesis in motor function
Several studies have investigated the significance of myelination for motor function. McKenzie et al. first reported OPC proliferation and generation of new myelinating oligodendrocytes in animals learning the complex wheel motor task [40, 41]. Importantly, these studies used genetic tools to block the generation of new oligodendrocytes and demonstrated that the activity-induced oligodendrogenesis and myelination are necessary components for motor learning and skill acquisition, especially in early stages of the learning paradigm [40, 41]. Consistent with these findings, Simon et al. also reported that voluntary exercise (i.e., wheel running) increased the cell cycle exit and consequently the differentiation of OPCs into myelinating oligodendrocytes [52]. In a more recent study, Bacmeister et al. investigated whether myelination is dynamically altered on individual cortical axons during motor learning. They used a forelimb reaching task assay and longitudinal in vivo two-photon imaging of the primary motor cortex. They observed increased sheath retraction during training, followed by increased sheath addition in the days after training [53]. Their study thus demonstrated that motor learning induces dynamic changes in myelination specifically on behaviorally activated axons, which correlates with motor performance [53]. Kato et al. investigated for myelination dependent changes in neural activity in motor cortex from motor learning using a genetic model with myelination deficiency caused by extra copies of the myelin proteolipid protein 1 gene [54]. They noted that deficit in myelin impaired motor learning was associated in variability in axonal conduction from thalamocortical axons, which results in variable cortical responses. From these observations, they concluded that myelin regulation helps to maintain the synchrony of cortical spike-time arrivals through long-range axons, facilitating the propagation of the information required for learning [54]. Therefore, activity-induced myelination resulting from motor learning is an important mechanism for remodeling neural circuits [55, 56]. Parallel to these demonstrations of the role of myelination in motor learning using rodent models, several studies using MRI technique diffusion tensor imaging (DTI) have reported measurable changes in WM from motor activity in humans. These studies include measurement of WM using DTI from piano practicing [57], juggling [58] and whole-body movement paradigm quadrato motor training (QMT) [59]. Motor learning also induces WM changes in rodent models learning a highly skilled reaching task [60]. Further details describing WM changes from motor activity have been described in depth elsewhere [4].
What is the mechanism by which motor activity promotes myelination? While it is known that myelination facilitates the propagation of action potentials in axons, the reciprocal impact of neuronal activity on the cellular state of oligodendrocytes is often overlooked. Seminal experiments by Barres et al. demonstrated that neuronal activity play a role in controlling the number of oligodendrocytes. Pharmacological blockade of action potentials in the retina inhibits OPC proliferation in optic nerves [61]. Other studies have reiterated these findings, showing that electrical stimulation of medullary pyramids promotes the proliferation and differentiation of OPCs in the corticospinal tract of adult rats [62]. Similarly, optogenetic stimulation of cortical layer 5 (L5) excitatory neurons promote the proliferation and differentiation of OPCs [63]. Further details describing the role of neuronal activity on oligodendrogenesis have been described in depth elsewhere [64]. Thus, the generation of myelinating oligodendrocytes is promoted by motor function induced neuronal activity resulting in increased axon-glia interactions. These observations provide a clear hypothesis as to how THAP1 and its co-regulators may impact motor function based on their role in generating myelinating oligodendrocytes.
Onset of DYT6 and myelination during development
The onset of symptoms in DYT-THAP1 is consistent with neurodevelopmental abnormalities. Domingo et al. utilized literature sources and the ClinVar database to examine case reports of individuals and families with THAP1 gene-related dystonia [31]. They reported that 69.2% of cases displayed symptoms before the age of 20, with 82.6% of those experiencing onset before the age of 15, presenting with generalized or multifocal dystonia [31]. In a parallel study, Lange et al. conducted an extensive investigation using the MDSGene protocol, reviewing approximately 1,200 citations [32]. They analyzed 241 THAP1 mutation carriers from 169 families and determined a median age of onset at 15 years. Notably, 34.9% of patients experienced childhood onset, and 69.3% developed symptoms in early adulthood [32]. Thus, the onset of symptoms in DYT6 predominantly occurs in early ages, implicating neurodevelopmental events in its neuropathology.
The early developmental periods of childhood and adolescence are also marked by myelination of the white matter, which occurs in a non-linear manner [65]. The most rapid brain growth occurs during the first 3 years of life, accompanied by myelination primarily within the first 2 years in humans and the first 3 weeks in rodents [46]. The timing of myelination in cerebral white matter coincides with the developmental trajectories of cognitive and motor skills [66]. Multiple studies employing various approaches have concluded that the rate and extent of cortical myelination during development occur earlier in motor and sensory regions. In a comprehensive study involving 484 participants, Grydeland et al. explored the trajectory of myelination across the cortex in relation to age [67]. Peak myelination exhibited a bimodal distribution relative to age, with a pre-pubertal wave of myelination observed in primary sensory and motor cortices. A similar conclusion was drawn in one of the most well-defined descriptions of human myeloarchitecture, based on the findings of Oscar and Cecile Vogt by Rudolf Nieuwenhuys [66, 68]. These studies on the parcellation of the cortex describe that primary brain areas, both sensory and motor, show a greater degree of myelination, while the superior parietal cortex and prefrontal cortex have strikingly fewer myelinated axons. Thus, critical periods during the emergence of motor function coincide with the onset of DYT6 symptom and de novo myelination of the sensory and motor cortex.
Discussion
Unbiased transcriptomic studies using multiple DYT-THAP1 models has yielded valuable insights into a transcriptional regulatory network driven by THAP1 and its role in various cellular pathways. While the THAP1 regulated transcriptome is diverse and cell-type specific, it is bound and co-regulated by a common set of proteins which prominently target the axon-glia interactions governing myelination pathway at a molecular level. The importance of myelination in motor function as a neurodevelopmental process and also in its role in activity dependent remodeling of motor circuits, make it an important downstream target pathway relevant for dystonia. Further investigation of THAP1-mediated myelination and its impact on motor function will help us comprehend the contribution of axon-glia interactions in dystonia pathophysiology and provide opportunities for therapeutic interventions targeting these pathways.
Author contributions
DY wrote and edited the manuscript.
Funding
This research was supported in part by the Dystonia Medical Research Foundation DMRF-MCMD-2022-2 (to DY), National Institute of Neurological Disorders and Stroke R01NS122990 (to DY).
Conflict of interest
The author declares that the research was conducted in the absence of any commercial or financial relationships that could be construed as a potential conflict of interest.
Acknowledgments
We thank Dr. Cathy Collins for critical reading of the manuscript.
References
1. Albanese, A, Bhatia, K, Bressman, SB, Delong, MR, Fahn, S, Fung, VS, et al. Phenomenology and classification of dystonia: a consensus update. Mov Disord (2013) 28(7):863–73. doi:10.1002/mds.25475
2. Grutz, K, and Klein, C. Dystonia updates: definition, nomenclature, clinical classification, and etiology. J Neural Transm (2021) 128(4):395–404. doi:10.1007/s00702-021-02314-2
3. Fuchs, T, Gavarini, S, Saunders-Pullman, R, Raymond, D, Ehrlich, ME, Bressman, SB, et al. Mutations in the THAP1 gene are responsible for DYT6 primary torsion dystonia. Nat Genet (2009) 41(3):286–8. doi:10.1038/ng.304
4. Yellajoshyula, D, Pappas, SS, and Dauer, WT. Oligodendrocyte and extracellular matrix contributions to central nervous system motor function: implications for dystonia. Mov Disord (2022) 37(3):456–63. doi:10.1002/mds.28892
5. Roussigne, M, Cayrol, C, Clouaire, T, Amalric, F, and Girard, JP. THAP1 is a nuclear proapoptotic factor that links prostate-apoptosis-response-4 (Par-4) to PML nuclear bodies. Oncogene (2003) 22(16):2432–42. doi:10.1038/sj.onc.1206271
6. Cayrol, C, Lacroix, C, Mathe, C, Ecochard, V, Ceribelli, M, Loreau, E, et al. The THAP-zinc finger protein THAP1 regulates endothelial cell proliferation through modulation of pRB/E2F cell-cycle target genes. Blood (2007) 109(2):584–94. doi:10.1182/blood-2006-03-012013
7. Yellajoshyula, D, Liang, CC, Pappas, SS, Penati, S, Yang, A, Mecano, R, et al. The DYT6 dystonia protein THAP1 regulates myelination within the oligodendrocyte lineage. Dev Cel (2017) 42(1):52–67. doi:10.1016/j.devcel.2017.06.009
8. Frederick, NM, Shah, PV, Didonna, A, Langley, MR, Kanthasamy, AG, and Opal, P. Loss of the dystonia gene Thap1 leads to transcriptional deficits that converge on common pathogenic pathways in dystonic syndromes. Hum Mol Genet (2019) 28(8):1343–56. doi:10.1093/hmg/ddy433
9. Zakirova, Z, Fanutza, T, Bonet, J, Readhead, B, Zhang, W, Yi, Z, et al. Mutations in THAP1/DYT6 reveal that diverse dystonia genes disrupt similar neuronal pathways and functions. Plos Genet (2018) 14(1):e1007169. doi:10.1371/journal.pgen.1007169
10. Aguilo, F, Zakirova, Z, Nolan, K, Wagner, R, Sharma, R, Hogan, M, et al. THAP1: role in mouse embryonic stem cell survival and differentiation. Stem Cel Rep (2017) 9:92–107. doi:10.1016/j.stemcr.2017.04.032
11. Domingo, A, Yadav, R, Shah, S, Hendriks, WT, Erdin, S, Gao, D, et al. Dystonia-specific mutations in THAP1 alter transcription of genes associated with neurodevelopment and myelin. Am J Hum Genet (2021) 108(11):2145–58. doi:10.1016/j.ajhg.2021.09.017
12. Baumann, H, Ott, F, Weber, J, Trilck-Winkler, M, Munchau, A, Zittel, S, et al. Linking penetrance and transcription in DYT-THAP1: insights from a human iPSC-derived cortical model. Mov Disord (2021) 36(6):1381–91. doi:10.1002/mds.28506
13. Cheng, F, Zheng, W, Barbuti, PA, Bonsi, P, Liu, C, Casadei, N, et al. DYT6 mutated THAP1 is a cell type dependent regulator of the SP1 family. Brain (2022) 145(11):3968–84. doi:10.1093/brain/awac001
14. Yellajoshyula, D, Pappas, SS, Rogers, AE, Choudhury, B, Reed, X, Ding, J, et al. THAP1 modulates oligodendrocyte maturation by regulating ECM degradation in lysosomes. Proc Natl Acad Sci U S A (2021) 118(31):e2100862118. doi:10.1073/pnas.2100862118
15. Shinoda, K, Zong, D, Callen, E, Wu, W, Dumitrache, LC, Belinky, F, et al. The dystonia gene THAP1 controls DNA double-strand break repair choice. Mol Cel (2021) 81(12):2611–24.e10. doi:10.1016/j.molcel.2021.03.034
16. Myers, RM, Stamatoyannopoulos, J, Snyder, M, Dunham, I, Hardison, RC, Bernstein, BE, et al. A user's guide to the encyclopedia of DNA elements (ENCODE). Plos Biol (2011) 9(4):e1001046. doi:10.1371/journal.pbio.1001046
17. Staege, S, Kutschenko, A, Baumann, H, Glass, H, Henkel, L, Gschwendtberger, T, et al. Reduced expression of gaba A receptor Alpha2 subunit is associated with disinhibition of DYT-THAP1 dystonia patient-derived striatal medium spiny neurons. Front Cel Dev Biol (2021) 9:650586. doi:10.3389/fcell.2021.650586
18. Cheng, F, Walter, M, Wassouf, Z, Hentrich, T, Casadei, N, Schulze-Hentrich, J, et al. Unraveling molecular mechanisms of THAP1 missense mutations in DYT6 dystonia. J Mol Neurosci (2020) 70(7):999–1008. doi:10.1007/s12031-020-01490-2
19. Yellajoshyula, D, Rogers, AE, Kim, AJ, Kim, S, Pappas, SS, and Dauer, WT. A pathogenic DYT-THAP1 dystonia mutation causes hypomyelination and loss of YY1 binding. Hum Mol Genet (2022) 31(7):1096–104. doi:10.1093/hmg/ddab310
20. Pabian-Jewula, S, Bragiel-Pieczonka, A, and Rylski, M. Ying Yang 1 engagement in brain pathology. J Neurochem (2022) 161(3):236–53. doi:10.1111/jnc.15594
21. Carminho-Rodrigues, MT, Steel, D, Sousa, SB, Brandt, G, Guipponi, M, Laurent, S, et al. Complex movement disorder in a patient with heterozygous YY1 mutation (Gabriele-de Vries syndrome). Am J Med Genet A (2020) 182:2129–32. doi:10.1002/ajmg.a.61731
22. Ferng, A, Thulin, P, Walsh, E, Weissbrod, PA, and Friedman, J. YY1: a new gene for childhood onset dystonia with prominent oromandibular-laryngeal involvement? Mov Disord (2021) 37:227–8. doi:10.1002/mds.28813
23. Malaquias, MJ, Damasio, J, Mendes, A, Freixo, JP, and Magalhaes, M. A case of YY1-related isolated dystonia with severe oromandibular involvement. Mov Disord (2021) 36:2705–6. doi:10.1002/mds.28771
24. Zorzi, G, Keller Sarmiento, IJ, Danti, FR, Bustos, BI, Invernizzi, F, Panteghini, C, et al. YY1-Related dystonia: clinical aspects and long-term response to deep brain stimulation. Mov Disord (2021) 36(6):1461–2. doi:10.1002/mds.28547
25. Hollstein, R, Reiz, B, Kotter, L, Richter, A, Schaake, S, Lohmann, K, et al. Dystonia-causing mutations in the transcription factor THAP1 disrupt HCFC1 cofactor recruitment and alter gene expression. Hum Mol Genet (2017) 26(15):2975–83. doi:10.1093/hmg/ddx187
26. Mazars, R, Gonzalez-de-Peredo, A, Cayrol, C, Lavigne, AC, Vogel, JL, Ortega, N, et al. The THAP-zinc finger protein THAP1 associates with coactivator HCF-1 and O-GlcNAc transferase: a link between DYT6 and DYT3 dystonias. J Biol Chem (2010) 285(18):13364–71. doi:10.1074/jbc.M109.072579
27. Dulovic-Mahlow, M, Gajos, A, Baumann, H, Pozojevic, J, Kaiser, FJ, Bogucki, A, et al. Highly reduced penetrance in a family with a THAP1 nonsense mutation: role of THAP1 expression? Parkinsonism Relat Disord (2019) 65:274–6. doi:10.1016/j.parkreldis.2019.05.036
28. Erogullari, A, Hollstein, R, Seibler, P, Braunholz, D, Koschmidder, E, Depping, R, et al. THAP1, the gene mutated in DYT6 dystonia, autoregulates its own expression. Biochim Biophys Acta (2014) 1839(11):1196–204. doi:10.1016/j.bbagrm.2014.07.019
29. Blanchard, A, Ea, V, Roubertie, A, Martin, M, Coquart, C, Claustres, M, et al. DYT6 dystonia: review of the literature and creation of the UMD Locus-Specific Database (LSDB) for mutations in the THAP1 gene. Hum Mutat (2011) 32(11):1213–24. doi:10.1002/humu.21564
30. Bragg, DC, Armata, IA, Nery, FC, Breakefield, XO, and Sharma, N. Molecular pathways in dystonia. Neurobiol Dis (2011) 42(2):136–47. doi:10.1016/j.nbd.2010.11.015
31. Domingo, A, Yadav, R, and Ozelius, LJ. Isolated dystonia: clinical and genetic updates. J Neural Transm (Vienna) (2020) 128:405–16. doi:10.1007/s00702-020-02268-x
32. Lange, LM, Junker, J, Loens, S, Baumann, H, Olschewski, L, Schaake, S, et al. Genotype-phenotype relations for isolated dystonia genes: MDSGene systematic review. Mov Disord (2021) 36(5):1086–103. doi:10.1002/mds.28485
33. Clouaire, T, Roussigne, M, Ecochard, V, Mathe, C, Amalric, F, and Girard, JP. The THAP domain of THAP1 is a large C2CH module with zinc-dependent sequence-specific DNA-binding activity. Proc Natl Acad Sci USA (2005) 102(19):6907–12. doi:10.1073/pnas.0406882102
34. Bessiere, D, Lacroix, C, Campagne, S, Ecochard, V, Guillet, V, Mourey, L, et al. Structure-function analysis of the THAP zinc finger of THAP1, a large C2CH DNA-binding module linked to Rb/E2F pathways. J Biol Chem (2008) 283(7):4352–63. doi:10.1074/jbc.M707537200
35. Campagne, S, Saurel, O, Gervais, V, and Milon, A. Structural determinants of specific DNA-recognition by the THAP zinc finger. Nucleic Acids Res (2010) 38(10):3466–76. doi:10.1093/nar/gkq053
36. Sengel, C, Gavarini, S, Sharma, N, Ozelius, LJ, and Bragg, DC. Dimerization of the DYT6 dystonia protein, THAP1, requires residues within the coiled-coil domain. J Neurochem (2011) 118(6):1087–100. doi:10.1111/j.1471-4159.2011.07386.x
37. Diaw, SH, Ott, F, Münchau, A, Lohmann, K, and Busch, H. Emerging role of a systems biology approach to elucidate factors of reduced penetrance: transcriptional changes in THAP1-linked dystonia as an example. Medizinische Genetik (2022) 34(2):131–41. doi:10.1515/medgen-2022-2126
38. He, Y, Dupree, J, Wang, J, Sandoval, J, Li, J, Liu, H, et al. The transcription factor Yin Yang 1 is essential for oligodendrocyte progenitor differentiation. Neuron (2007) 55(2):217–30. doi:10.1016/j.neuron.2007.06.029
39. Tretiakova, A, Steplewski, A, Johnson, EM, Khalili, K, and Amini, S. Regulation of myelin basic protein gene transcription by Sp1 and Puralpha: evidence for association of Sp1 and Puralpha in brain. J Cel Physiol (1999) 181(1):160–8. doi:10.1002/(SICI)1097-4652(199910)181:1<160::AID-JCP17>3.0.CO;2-H
40. McKenzie, IA, Ohayon, D, Li, H, de Faria, JP, Emery, B, Tohyama, K, et al. Motor skill learning requires active central myelination. Science (2014) 346(6207):318–22. doi:10.1126/science.1254960
41. Xiao, L, Ohayon, D, McKenzie, IA, Sinclair-Wilson, A, Wright, JL, Fudge, AD, et al. Rapid production of new oligodendrocytes is required in the earliest stages of motor-skill learning. Nat Neurosci (2016) 19(9):1210–7. doi:10.1038/nn.4351
42. Bacmeister, CM, Barr, HJ, McClain, CR, Thornton, MA, Nettles, D, Welle, CG, et al. Motor learning promotes remyelination via new and surviving oligodendrocytes. Nat Neurosci (2020) 23(7):819–31. doi:10.1038/s41593-020-0637-3
43. Waxman, SG, and Bennett, MV. Relative conduction velocities of small myelinated and non-myelinated fibres in the central nervous system. Nat New Biol (1972) 238(85):217–9. doi:10.1038/newbio238217a0
44. Semple, BD, Blomgren, K, Gimlin, K, Ferriero, DM, and Noble-Haeusslein, LJ. Brain development in rodents and humans: identifying benchmarks of maturation and vulnerability to injury across species. Prog Neurobiol (2013) 106-107:1–16. doi:10.1016/j.pneurobio.2013.04.001
45. Keshavan, MS, Diwadkar, VA, DeBellis, M, Dick, E, Kotwal, R, Rosenberg, DR, et al. Development of the corpus callosum in childhood, adolescence and early adulthood. Life Sci (2002) 70(16):1909–22. doi:10.1016/s0024-3205(02)01492-3
46. Rice, D, and Barone, S. Critical periods of vulnerability for the developing nervous system: evidence from humans and animal models. Environ Health Perspect (2000) 108(3):511–33. doi:10.1289/ehp.00108s3511
47. Lohmann, K, Uflacker, N, Erogullari, A, Lohnau, T, Winkler, S, Dendorfer, A, et al. Identification and functional analysis of novel THAP1 mutations. Eur J Hum Genet (2012) 20(2):171–5. doi:10.1038/ejhg.2011.159
48. Guo, L, Eviatar-Ribak, T, and Miskimins, R. Sp1 phosphorylation is involved in myelin basic protein gene transcription. J Neurosci Res (2010) 88(15):3233–42. doi:10.1002/jnr.22486
49. Paez, PM, Garcia, CI, and Pasquini, JM. Expression of myelin basic protein in two oligodendroglial cell lines is modulated by apotransferrin through different transcription factors. J Neurosci Res (2006) 83(4):606–18. doi:10.1002/jnr.20750
50. Wei, Q, Miskimins, WK, and Miskimins, R. Stage-specific expression of myelin basic protein in oligodendrocytes involves Nkx2.2-mediated repression that is relieved by the Sp1 transcription factor. J Biol Chem (2005) 280(16):16284–94. doi:10.1074/jbc.M500491200
51. Lehericy, S, Tijssen, MA, Vidailhet, M, Kaji, R, and Meunier, S. The anatomical basis of dystonia: current view using neuroimaging. Mov Disord (2013) 28(7):944–57. doi:10.1002/mds.25527
52. Simon, C, Gotz, M, and Dimou, L. Progenitors in the adult cerebral cortex: cell cycle properties and regulation by physiological stimuli and injury. Glia (2011) 59(6):869–81. doi:10.1002/glia.21156
53. Bacmeister, CM, Huang, R, Osso, LA, Thornton, MA, Conant, L, Chavez, AR, et al. Motor learning drives dynamic patterns of intermittent myelination on learning-activated axons. Nat Neurosci (2022) 25(10):1300–13. doi:10.1038/s41593-022-01169-4
54. Kato, D, Wake, H, Lee, PR, Tachibana, Y, Ono, R, Sugio, S, et al. Motor learning requires myelination to reduce asynchrony and spontaneity in neural activity. Glia (2020) 68(1):193–210. doi:10.1002/glia.23713
55. Stevens, B, Tanner, S, and Fields, RD. Control of myelination by specific patterns of neural impulses. J Neurosci (1998) 18(22):9303–11. doi:10.1523/JNEUROSCI.18-22-09303.1998
56. Wake, H, Lee, PR, and Fields, RD. Control of local protein synthesis and initial events in myelination by action potentials. Science (2011) 333(6049):1647–51. doi:10.1126/science.1206998
57. Bengtsson, SL, Nagy, Z, Skare, S, Forsman, L, Forssberg, H, and Ullen, F. Extensive piano practicing has regionally specific effects on white matter development. Nat Neurosci (2005) 8(9):1148–50. doi:10.1038/nn1516
58. Scholz, J, Klein, MC, Behrens, TE, and Johansen-Berg, H. Training induces changes in white-matter architecture. Nat Neurosci (2009) 12(11):1370–1. doi:10.1038/nn.2412
59. Piervincenzi, C, Ben-Soussan, TD, Mauro, F, Mallio, CA, Errante, Y, Quattrocchi, CC, et al. White matter microstructural changes following quadrato motor training: a longitudinal study. Front Hum Neurosci (2017) 11:590. doi:10.3389/fnhum.2017.00590
60. Sampaio-Baptista, C, Khrapitchev, AA, Foxley, S, Schlagheck, T, Scholz, J, Jbabdi, S, et al. Motor skill learning induces changes in white matter microstructure and myelination. J Neurosci (2013) 33(50):19499–503. doi:10.1523/JNEUROSCI.3048-13.2013
61. Barres, BA, and Raff, MC. Proliferation of oligodendrocyte precursor cells depends on electrical activity in axons. Nature (1993) 361(6409):258–60. doi:10.1038/361258a0
62. Li, Q, Brus-Ramer, M, Martin, JH, and McDonald, JW. Electrical stimulation of the medullary pyramid promotes proliferation and differentiation of oligodendrocyte progenitor cells in the corticospinal tract of the adult rat. Neurosci Lett (2010) 479(2):128–33. doi:10.1016/j.neulet.2010.05.043
63. Gibson, EM, Purger, D, Mount, CW, Goldstein, AK, Lin, GL, Wood, LS, et al. Neuronal activity promotes oligodendrogenesis and adaptive myelination in the mammalian brain. Science (2014) 344(6183):1252304. doi:10.1126/science.1252304
64. Kato, D, and Wake, H. Activity-dependent myelination. Adv Exp Med Biol (2019) 1190:43–51. doi:10.1007/978-981-32-9636-7_4
65. Buyanova, IS, and Arsalidou, M. Cerebral white matter myelination and relations to age, gender, and cognition: a selective review. Front Hum Neurosci (2021) 15:662031. doi:10.3389/fnhum.2021.662031
66. Turner, R. Myelin and modeling: bootstrapping cortical microcircuits. Front Neural Circuits (2019) 13:34. doi:10.3389/fncir.2019.00034
67. Grydeland, H, Vertes, PE, Vasa, F, Romero-Garcia, R, Whitaker, K, Alexander-Bloch, AF, et al. Waves of maturation and senescence in micro-structural MRI markers of human cortical myelination over the lifespan. Cereb Cortex (2019) 29(3):1369–81. doi:10.1093/cercor/bhy330
Keywords: dystonia, myelination, THAP1, white matter, transcription, YY1
Citation: Yellajoshyula D (2023) Transcriptional regulatory network for neuron-glia interactions and its implication for DYT6 dystonia. Dystonia 2:11796. doi: 10.3389/dyst.2023.11796
Received: 13 July 2023; Accepted: 17 October 2023;
Published: 30 October 2023.
Edited by:
Aasef Shaikh, Case Western Reserve University, United StatesCopyright © 2023 Yellajoshyula. This is an open-access article distributed under the terms of the Creative Commons Attribution License (CC BY). The use, distribution or reproduction in other forums is permitted, provided the original author(s) and the copyright owner(s) are credited and that the original publication in this journal is cited, in accordance with accepted academic practice. No use, distribution or reproduction is permitted which does not comply with these terms.
*Correspondence: Dhananjay Yellajoshyula, ZHh5MjcwQGNhc2UuZWR1