- 1Department of Neurology, Norman Fixel Institute of Neurological Diseases, McKnight Brain Institute, College of Medicine, University of Florida, Gainesville, FL, United States
- 2Department of Applied Physiology and Kinesiology, University of Florida, Gainesville, FL, United States
DYT1 dystonia is an inherited early-onset generalized dystonia characterized by sustained muscle contractions causing abnormal, repetitive movements or postures. Most DYT1 patients have a heterozygous trinucleotide GAG deletion (ΔGAG) in DYT1/TOR1A, coding for torsinA. Dyt1 heterozygous ΔGAG knock-in (KI) mice or global KI mice show motor deficits and abnormal Purkinje cell firing. However, Purkinje cell-specific heterozygous ΔGAG conditional KI mice (Pcp2-KI) show improved motor performance, reduced sensory-evoked brain activation in the striatum and midbrain, and reduced functional connectivity of the striatum with the anterior medulla. Whether Pcp2-KI mice show similar abnormal Purkinje cell firing as the global KI mice, suggesting a cell-autonomous effect causes the abnormal Purkinje cell firing in the global KI mice, is unknown. We used acute cerebellar slice recording in Pcp2-KI mice to address this issue. The Pcp2-KI mice exhibited no changes in spontaneous firing and intrinsic excitability compared to the control mice. While membrane properties were largely unchanged, the resting membrane potential was slightly hyperpolarized, which was associated with decreased baseline excitability. Our results suggest that the abnormal Purkinje cell firing in the global KI mice was not cell-autonomous and was caused by physiological changes elsewhere in the brain circuits. Our results also contribute to the ongoing research of how basal ganglia and cerebellum interact to influence motor control in normal states and movement disorders.
Introduction
Dystonia is a movement disorder characterized by sustained or intermittent muscle contractions causing abnormal, often repetitive, movements or postures [1]. Dystonia can be caused by genetic mutations, brain injury, or the side effects of drugs. DYT1 dystonia is the most common type of early-onset generalized dystonia [2] with symptom onset from 5 to 28 years old. The majority of the individuals affected by DYT1 dystonia share a trinucleotide deletion (ΔGAG) located in the exon 5 of DYT1 or TOR1A gene, leading to a loss of a glutamate amino acid residue for torsinA (torsinA∆E) [3]. It is transmitted as an autosomal dominant disorder with a 30%–40% penetrance. Affected individuals could be seriously disabled and need to use a wheelchair. There are other types of isolated mutations found in DYT1 dystonia patients with missense changes at E121K, V129L, D194V, F205I, and R288Q [4–8], and three other deletions of an 18 bp DNA fragment deletion, a frame-shift mutation caused by 4 bp deletion, and a 6 bp deletion [8–10]. The latest epidemiology data indicate between 54,366 and 80,891 ΔGAG mutation carriers in the United States, including 16,475-24,513 DYT1 patients due to the reduced penetrance [11].
Animal models have been used to investigate the pathophysiology of genetic diseases and contribute to developing effective treatments. Multiple animal models have been generated for DYT1 dystonia [12–18]. Dyt1 KI mice have the corresponding in-frame trinucleotide ∆GAG deletion mutation in the endogenous Dyt1 or Tor1a and model DYT1 ΔGAG patients [19, 20]. The Dyt1 KI mouse also shows dystonia-like phenotypes, including motor and sensory deficits, abnormal gait, and muscle co-contraction of the hind limbs [20–23]. A new conditional knock-in locus of Dyt1 or Tor1a (referred to as SWAP) was developed, possessing loxP sites flanking exon 5 of the Tor1a gene, along with an additional downstream mutant exon 5 containing a ΔGAG knock-in mutation [24]. When crossed with Pcp2-cre mice [25], the WT exon five was deleted, and the mutant ΔGAG was expressed specifically in Purkinje cells. The resulting conditional knock-in mice, referred to as Pcp2-KI mice, show improved motor performance, reduced sensory-evoked brain activation in the striatum and midbrain, and reduced striatum functional connectivity with the anterior medulla [26].
Cerebellar circuits, especially Purkinje cells, are essential players in movement and posture control, and there are multiple lines of investigations implicating their involvement in dystonia pathogenesis [16, 27–37]. The shRNA-mediated knockdown of torsinA in wild-type mice leads to overt dystonic-like movements with cell death in the deep cerebellar nuclei [38]. Dyt1 KI mice show altered Purkinje cell morphology [39, 40] and firing, with increased large-conductance calcium-activated potassium (BK) current and the BK channel protein levels [41]. The abnormal function of cerebellar circuits is likely involved in the pathogenesis of DYT1 and other dystonias. However, whether the Pcp2-KI mice show similar altered Purkinje cell firing is unknown. Furthermore, the relative contribution of the striatum and cerebellum in the pathogenesis of DYT1 dystonia is unclear. Here, the Purkinje cells in the Pcp2-KI mice were characterized by electrophysiological recording of acute brain slices. The spontaneous firing, intrinsic excitability, and membrane properties of Purkinje cells were examined.
Materials and methods
Animals
All experiments complied with the United States Public Health Service Guide for Care and Use of Laboratory Animals and approved by the Institutional Animal Care and Use Committees of the University of Florida. Pcp2-KI mice and their littermate control mice were prepared and genotyped by PCR as described previously [26]. Pcp2-cre heterozygous mice [25] (The Jackson Laboratory strain #: 010536) were mated with Tor1aswap heterozygous mice [24] (The Jackson Laboratory strain #: 028099) to produce Pcp2-KI (Pcp2-cre+/−Tor1aswap+/−) and control mice (Pcp2-cre+/−). The presence of cre was detected with primers creF: 5′-CAGCTAAACATGCTTCATCGTC and creR: 5′-GTTATTCGGATCATCAGCTACACC. Tor1aswap allele was determined by primers 27427: 5′-TCCTCCCCCAAGTACATCAG and 27428: 5′-CATAGCTCAGCCGTCCAGTC [24]. Mice were housed under a 12-h light and 12-h dark cycle with ad libitum access to food and water. All experiments and initial data analysis were performed by investigators blind to the genotypes. This study followed the recommended heterogenization of study samples of various ages, and the data were analyzed with age as a covariate [42].
Brain slice electrophysiology
Electrophysiological recordings and data analysis for spontaneous firing, intrinsic excitability, and membrane properties of 128 Purkinje cells were obtained from 9 control and 7 Pcp2-KI littermate male mice (212–385 days old), as described previously [41, 43–45]. Since the onset of motor deficits is about 6.5 months old in the case of Dyt1 KI mice [20, 46], mice older than the onset age were used in the present study. Briefly, the cell-attached recordings of Purkinje cells were performed in the parasagittal 300 μm-thick cerebellar brain slices. After recording the spontaneous firing, whole-cell recordings were made by breaking through the membrane. The electrophysiological intrinsic membrane properties (resting membrane potential, capacitance, membrane resistance, and time constant) were measured in the whole-cell recording mode. The current steps were injected, and the evoked-action potentials were recorded. We used male mice to minimize the variation due to the estrous cycle and to match the sex we used in our previous study for direct comparison [41].
Statistics
Data were tested for normality first using the univariate procedure of the SAS statistical package. A generalized linear model (GENMOD) was used to compare the spontaneous firing, intrinsic excitability, and membrane properties. Age was used as a continuous variable, and data from each cell were nested within animals and treated as repeated measurements. A negative binomial distribution was used for count data, i.e., the number of action potentials in the current injection. A gamma distribution was used for data that was not normally distributed. For tonic/non-tonic cell distribution analysis, chi-square was used. Significance was assigned at p < 0.05. Data in the text are presented as “mean ± standard error of the mean (SEM)” unless specified otherwise.
Results
Normal spontaneous firing frequency, coefficient of variation (CV), and cell type distribution of the Purkinje cells in Pcp2-KI mice
Cerebellar Purkinje cells are the sole output of the cerebellum and play an essential role in cerebellar function. The Purkinje cells in the Pcp2-KI mice were characterized by acute brain slice recording. The spontaneous firing of the Purkinje cells was recorded by cell-attached recording mode with a voltage clamp (control, 61 cells/9 mice; Pcp2-KI, 35 cells/7 mice). The representative traces of the Purkinje cells were shown in Figure 1A. Neither the firing frequency (control, 39.6 ± 7.5 Hz; Pcp2-KI, 32.1 ± 2.0; p = 0.30, Figure 1B) nor CV (control, 0.254 ± 0.012; Pcp2-KI, 0.261 ± 0.021; p = 0.77; Figure 1C) was significantly altered in Pcp2-KI mice compared to control mice. Purkinje cells can be grouped into tonic and non-tonic types [41, 44, 47]. When analyzed separately by the cell types, neither the firing frequency nor CV was altered in both cell types (Table 1). Finally, the relative ratio of the tonic and non-tonic cells was analyzed, and there was no significant difference between the control and Pcp2-KI mice (control: tonic = 35, non-tonic = 26; Pcp2-KI: tonic = 19, non-tonic = 16, p = 0.77). Overall, Pcp2-KI mice had normal spontaneous firing frequency, CV, and cell type distribution of Purkinje cells.
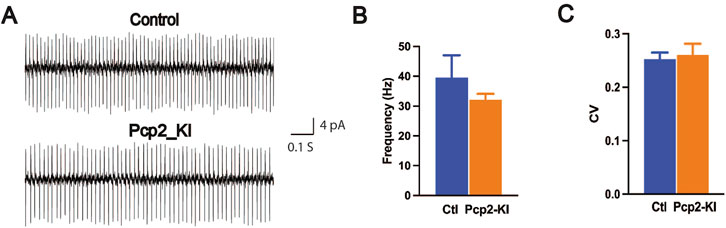
Figure 1. Spontaneous firing of the Purkinje cells in brain slices. (A) The representative traces of the Purkinje cells. Spontaneous firing frequency (B) and CV (C) were comparable between the control (Ctl) and Pcp2-KI mice. The bars represent means ± SEM.
Intrinsic excitability and membrane properties of the Purkinje cells in Pcp2-KI mice
After recording the spontaneous firing by cell-attached mode, the intrinsic membrane properties were measured in whole-cell recording mode. The resting membrane property of the Purkinje cells was determined from 9 control (73 cells) and 7 Pcp2-KI mice (55 cells). There was no significant difference in the membrane capacitance, the membrane resistance, or the time constant between the control and Pcp2-KI mice (Table 2). However, the resting membrane potential (RMP) of the Pcp2-KI mice was significantly hyperpolarized than that of the control mice, suggesting slightly decreased baseline excitability.
The intrinsic excitability of the Purkinje cells in the brain slices was measured with current step injections. The recorded neurons showed typical electrophysiological responses of the Purkinje cells (Figure 2A). The number of action potentials fired overall (control, 25.4 ± 2.3; Pcp2-KI, 26.1 ± 1.3; p = 0.78, Figure 2B) and at each current step (Figure 2C) were similar between control and Pcp2-KI mice. This indicates that while the neurons were less excitable at rest, their ability to respond to depolarizing stimuli was preserved, potentially reflecting compensatory mechanisms that maintained functional responsiveness despite altered baseline properties.
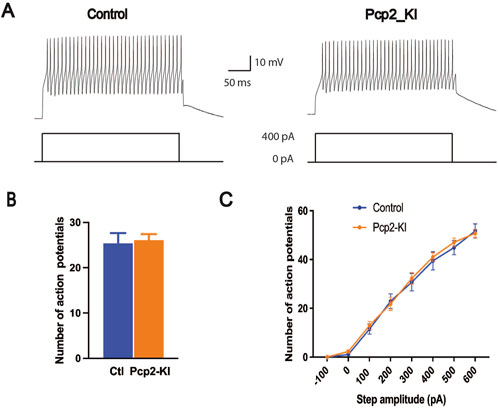
Figure 2. Intrinsic excitability of Purkinje cells as measured by current steps in the brain slices. Representative trace of the action potential firing in response to the current injection (A). The number of action potentials of all eight steps combined (B) and at each current step (C) was not significantly altered in Pcp2-KI mice compared to control (Ctl) mice. Means ± SEM were plotted.
Discussion
The current study aimed to determine whether abnormal Purkinje cell firing in the global KI mice is cell-autonomous using Pcp2-KI mice. The Pcp2-KI mice showed no changes in spontaneous firing, intrinsic excitability, and most membrane properties. The only subtle change we found was a slightly hyperpolarized resting membrane potential. These results suggest that the abnormal Purkinje cell firing in the global KI mice is not cell-autonomous and is driven by changes elsewhere in the brain circuits. The results are reminiscent of the mouse models of DYT11 dystonia. The Sgce global knockout mice showed nuclear envelope deficits, while Purkinje cell- or striatum-specific Sgce KO mice have no such deficits [48, 49].
Past studies have provided strong evidence that the cerebellum is actively involved in the pathogenesis of dystonia [16, 27–37]. However, it is unknown whether the dystonia originates from the basal ganglia, cerebellum, or both [32]. The overwhelming majority of the DYT1 patients have the ΔGAG mutation in the DYT1/TOR1A gene. Mutant torsinA could lead to both a loss of function and a toxic gain of function [50, 51]. Conditional knockout of torsinA in the striatum, the cerebral cortex, cholinergic interneurons, and dopamine receptor 1 or 2-positive neurons leads to motor deficits or overt dystonia [52–60]. Conditional knockin of mutant torsinA in dopamine receptor 2-positive neurons (D2-KI) leads to similar motor deficits [26]. These genetic experiments in animals suggest that mutations introduced in the basal ganglia circuit alone are sufficient to induce motor deficits or overt dystonia and support a basal ganglia origin for DYT1 dystonia.
On the other hand, it is remarkable that both conditional knockouts of torsinA (pKO) and conditional knockin of mutant torsinA (Pcp2-KI) in Purkinje cells show better motor performance in mice [26, 61]. The motor phenotype in pKO and Pcp2-KI mice suggests that torsinA mutations in Purkinje cells are insufficient to produce motor deficits. The normal Purkinje cell firing in Pcp2-KI mice extends these findings. It indicates mutant torsinA in Purkinje cells alone cannot induce abnormal Purkinje cell firing observed in the global KI mice. The behavioral and electrophysiological phenotypes in pKO and Pcp2-KI mice argue against a cerebellum origin for DYT1 dystonia. However, we can not rule out the possibility that in the global KI mice and DYT1 patients, the dystonia may still originate from Purkinje cells or other cerebellar neurons. This can be addressed with lines of Dyt1 conditional knockin mice that express wild-type torsinA in the Purkinje cells, other cerebellar neurons, or both.
Interestingly, acute shRNA-mediated torsinA knockdown in adult mice shows overt dystonic-like movements [38]. We generated an acute torsinA cerebellar knockdown mouse model by bilateral stereotaxic injections of AAV5-CMV-Cre-GFP into the cerebellum of Dyt1 loxP/loxP mice [57]. Expression of cre led to cre-loxP-mediated recombination and eliminated the expression of torsinA in AAV-infected cells. These mice showed overt dystonia similar to the shRNA-mediated torsinA knockdown mice (unpublished data). In addition, Purkinje cells in global KI mice show altered dendritic structure [39, 40] and altered spontaneous firing in vitro [41]. These results align with a cerebellum origin for DYT1 dystonia. Alternatively, the results could be interpreted as the cerebellum not acting as the origin but as a node downstream of the brain network abnormality that leads to the pathogenesis of DYT1 dystonia.
What might be the upstream brain network abnormality that drives Purkinje cell abnormality in DYT1 dystonia and global KI mice? The basal ganglia and the cerebellum are interconnected at the subcortical level with disynaptic pathways. The subthalamic nucleus in the basal ganglia connects to the cerebellar cortex via pontine nuclei [62]. Imaging studies in DYT1 mouse models indicate striatum alterations can influence cerebellar circuits. Forebrain torsinA knockout increases functional connectivity of the left striatum with the cerebellum [63]. Compared to controls, there is increased functional connectivity between the right dorsomedial striatum and the right cerebellar cortex in Dyt1 Ch2KO mice, with torsinA selectively knocked out in cholinergic neurons [59]. The Purkinje cell abnormality in Dyt1 KI mice [39–41] likely originated from the striatum. Future studies should analyze the Purkinje cell activity in the striatum-specific Dyt1 conditional knockout [56] or knockin mice to explore such a possibility.
The cerebellum can modulate the activity of the basal ganglia, especially the striatum. The dentate nucleus in the cerebellum connects to the striatum via the thalamus [62] or directly forms monosynaptic glutamatergic connections with the dopaminergic neurons in the substantia nigra (SN), which in turn, modulate the striatal activity [64, 65]. The current study uncovered normal Purkinje cell firing in Pcp2-KI mice, except for the slightly hyperpolarized RMP. There are limitations associated with the current study. We used glutamatergic and GABAergic antagonists to block synaptic transmission in brain slice recording. Synaptic inputs to Purkinje cells were not measured. Furthermore, we did not investigate whether altered RMP leads to any physiological changes elsewhere. However, brain imaging studies of Pcp2-KI mice show reduced sensory-evoked brain activation in the striatum and midbrain and reduced striatum functional connectivity with the anterior medulla [26]. These functional changes in the basal ganglia circuit may result from the Purkinje cell-specific knockin of the mutant torsinA. Although details are unclear, these changes are likely key to understanding improved motor performance in Pcp2-KI mice and, by extension, the pKO mice. We demonstrated earlier that Purkinje cell-specific knockout of torsinA in global Dyt1 KI mice (pKG mice) could alleviate the motor deficits associated with the Dyt1 KI mice [61]. Future studies focusing on the electrophysiological analysis of Purkinje cells, striatal medium spiny neurons, and SN dopaminergic neurons in pKO, pKG, and Pcp2-KI will clarify the roles of cerebellum and striatum in the pathogenesis of DYT1 dystonia and how to target these connections for novel treatments. These studies will have implications in basic neuroscience research beyond the dystonia field.
Data availability statement
The raw data supporting the conclusions of this article will be made available by the authors, without undue reservation.
Ethics statement
The animal study was approved by University of Florida Institutional Animal Care and Use Committee. The study was conducted in accordance with the local legislation and institutional requirements.
Author contributions
All authors participated in the design, interpretation of the studies, analysis of the data, and review of the manuscript; HX, PG, YnL, FY, and YqL conducted the experiments, and HX and YqL wrote the manuscript. All authors contributed to the article and approved the submitted version.
Funding
The author(s) declare that financial support was received for the research, authorship, and/or publication of this article. Research reported in this publication was provided by Tyler’s Hope for a Dystonia Cure and the Norman Fixel Institute for Neurological Diseases at UF Health, National Institutes of Health grants (NS75012, NS129873, and AG087418). The content is solely the authors’ responsibility and does not necessarily represent the official views of the National Institutes of Health. HX, FY, and YqL were partially supported by the Office of the Assistant Secretary of Defense for Health Affairs through the Peer-Reviewed Medical Research Program Discovery Award (W81XWH1810099 and W81XWH2110198). Opinions, interpretations, conclusions, and recommendations are those of the author and are not necessarily endorsed by the Department of Defense.
Acknowledgments
We thank the animal care staff and undergraduate students for their technical assistance.
Conflict of interest
The authors declare that the research was conducted in the absence of any commercial or financial relationships that could be construed as a potential conflict of interest.
Generative AI statement
The author(s) declare that no Generative AI was used in the creation of this manuscript.
Abbreviations
BK channel, large-conductance calcium-activated potassium channel; Ch2KO mice, cholinergic neuron-specific Dyt1 conditional knockout mice; CV, coefficient of variation; Dyt1 KI mice, Dyt1 ΔGAG heterozygous knock-in mice; KI, knockin; KO, knockout; Pcp2-KI, Purkinje cell-specific heterozygous ΔGAG conditional KI mice; PCR, polymerase chain reaction; pKG, Purkinje cell-specific knockout of torsinA in global Dyt1 KI mice; pKO, conditional knockouts of torsinA in Purkinje cells; RMP, resting membrane potential; SEM, standard error of the mean; SN, substantia nigra; SWAP, conditional knock-in locus of Dyt1 or Tor1a.
References
1. Albanese, A, Bhatia, K, Bressman, SB, DeLong, MR, Fahn, S, Fung, VS, et al. Phenomenology and classification of dystonia: a consensus update. Mov Disord (2013) 28:863–73. doi:10.1002/mds.25475
2. Bressman, SB, Sabatti, C, Raymond, D, de Leon, D, Klein, C, Kramer, PL, et al. The DYT1 phenotype and guidelines for diagnostic testing. Neurology (2000) 54:1746–52. doi:10.1212/wnl.54.9.1746
3. Klein, C, and Ozelius, LJ. Dystonia: clinical features, genetics, and treatment. Curr Opin Neurol (2002) 15:491–7. doi:10.1097/00019052-200208000-00014
4. Zirn, B, Grundmann, K, Huppke, P, Puthenparampil, J, Wolburg, H, Riess, O, et al. Novel TOR1A mutation p.Arg288Gln in early-onset dystonia (DYT1). J Neurol Neurosurg Psychiatry (2008) 79:1327–30. doi:10.1136/jnnp.2008.148270
5. Dobricic, V, Kresojevic, N, Zarkovic, M, Tomic, A, Marjanovic, A, Westenberger, A, et al. Phenotype of non-c.907_909delGAG mutations in TOR1A: DYT1 dystonia revisited. Parkinsonism Relat Disord (2015) 21:1256–9. doi:10.1016/j.parkreldis.2015.08.001
6. Calakos, N, Patel, VD, Gottron, M, Wang, G, Tran-Viet, KN, Brewington, D, et al. Functional evidence implicating a novel TOR1A mutation in idiopathic, late-onset focal dystonia. J Med Genet (2010) 47:646–50. doi:10.1136/jmg.2009.072082
7. Cheng, FB, Feng, JC, Ma, LY, Miao, J, Ott, T, Wan, XH, et al. Combined occurrence of a novel TOR1A and a THAP1 mutation in primary dystonia. Mov Disord (2014) 29:1079–83. doi:10.1002/mds.25921
8. Vulinovic, F, Lohmann, K, Rakovic, A, Capetian, P, Alvarez-Fischer, D, Schmidt, A, et al. Unraveling cellular phenotypes of novel TorsinA/TOR1A mutations. Hum Mutat (2014) 35:1114–22. doi:10.1002/humu.22604
9. Ritz, K, Groen, JL, Kruisdijk, JJ, Baas, F, Koelman, JH, and Tijssen, MA. Screening for dystonia genes DYT1, 11 and 16 in patients with writer's cramp. Mov Disord (2009) 24:1390–2. doi:10.1002/mds.22632
10. Leung, JC, Klein, C, Friedman, J, Vieregge, P, Jacobs, H, Doheny, D, et al. Novel mutation in the TOR1A (DYT1) gene in atypical early onset dystonia and polymorphisms in dystonia and early onset parkinsonism. Neurogenetics (2001) 3:133–43. doi:10.1007/s100480100111
11. Park, J, Damrauer, SM, Baras, A, Reid, JG, Overton, JD, and Gonzalez-Alegre, P. Epidemiology of DYT1 dystonia: estimating prevalence via genetic ascertainment. Neurol Genet (2019) 5:e358. doi:10.1212/NXG.0000000000000358
12. Oleas, J, Yokoi, F, DeAndrade, MP, Pisani, A, and Li, Y. Engineering animal models of dystonia. Mov Disord (2013) 28:990–1000. doi:10.1002/mds.25583
13. Richter, F, and Richter, A. Genetic animal models of dystonia: common features and diversities. Prog Neurobiol (2014) 121:91–113. doi:10.1016/j.pneurobio.2014.07.002
14. Tassone, A, Sciamanna, G, Bonsi, P, Martella, G, and Pisani, A. Experimental models of dystonia. Int Rev Neurobiol (2011) 98:551–72. doi:10.1016/B978-0-12-381328-2.00020-1
16. Rey Hipolito, AG, van der Heijden, ME, and Sillitoe, RV. Physiology of dystonia: animal studies. Int Rev Neurobiol (2023) 169:163–215. doi:10.1016/bs.irn.2023.05.004
17. El Atiallah, I, Bonsi, P, Tassone, A, Martella, G, Biella, G, Castagno, AN, et al. Synaptic dysfunction in dystonia: update from experimental models. Curr Neuropharmacol (2023) 21:2310–22. doi:10.2174/1570159X21666230718100156
18. Oleas, J, Yokoi, F, DeAndrade, MP, and Li, Y. In: M LeDoux, editor. Movement disorders: genetics and models. Academic Press. New York (2015), pp. 483–505.
19. Goodchild, RE, Kim, CE, and Dauer, WT. Loss of the dystonia-associated protein torsinA selectively disrupts the neuronal nuclear envelope. Neuron (2005) 48:923–32. doi:10.1016/j.neuron.2005.11.010
20. Dang, MT, Yokoi, F, McNaught, KS, Jengelley, TA, Jackson, T, Li, J, et al. Generation and characterization of Dyt1 DeltaGAG knock-in mouse as a model for early-onset dystonia. Exp Neurol (2005) 196:452–63. doi:10.1016/j.expneurol.2005.08.025
21. DeAndrade, MP, Trongnetrpunya, A, Yokoi, F, Cheetham, CC, Peng, N, Wyss, JM, et al. Electromyographic evidence in support of a knock-in mouse model of DYT1 Dystonia. Mov Disord (2016) 31:1633–9. doi:10.1002/mds.26677
22. Song, CH, Fan, X, Exeter, CJ, Hess, EJ, and Jinnah, HA. Functional analysis of dopaminergic systems in a DYT1 knock-in mouse model of dystonia. Neurobiol Dis (2012) 48:66–78. doi:10.1016/j.nbd.2012.05.009
23. Richter, F, Gerstenberger, J, Bauer, A, Liang, CC, and Richter, A. Sensorimotor tests unmask a phenotype in the DYT1 knock-in mouse model of dystonia. Behav Brain Res (2017) 317:536–41. doi:10.1016/j.bbr.2016.10.028
24. Weisheit, CE, and Dauer, WT. A novel conditional knock-in approach defines molecular and circuit effects of the DYT1 dystonia mutation. Hum Mol Genet (2015) 24:6459–72. doi:10.1093/hmg/ddv355
25. Zhang, XM, Ng, AH, Tanner, JA, Wu, WT, Copeland, NG, Jenkins, NA, et al. Highly restricted expression of Cre recombinase in cerebellar Purkinje cells. Genesis (2004) 40:45–51. doi:10.1002/gene.20062
26. Wilkes, BJ, Adury, RZ, Berryman, D, Concepcion, LR, Liu, Y, Yokoi, F, et al. Cell-specific Dyt1 ∆GAG knock-in to basal ganglia and cerebellum reveal differential effects on motor behavior and sensorimotor network function. Exp Neurol (2023) 367:114471. doi:10.1016/j.expneurol.2023.114471
27. Bologna, M, and Berardelli, A. The cerebellum and dystonia. Handb Clin Neurol (2018) 155:259–72. doi:10.1016/B978-0-444-64189-2.00017-2
28. Kaji, R, Bhatia, K, and Graybiel, AM. Pathogenesis of dystonia: is it of cerebellar or basal ganglia origin? J Neurol Neurosurg Psychiatry (2018) 89:488–92. doi:10.1136/jnnp-2017-316250
29. Shakkottai, VG, Batla, A, Bhatia, K, Dauer, WT, Dresel, C, Niethammer, M, et al. Current opinions and areas of consensus on the role of the cerebellum in dystonia. Cerebellum (2017) 16:577–94. doi:10.1007/s12311-016-0825-6
30. Tewari, A, Fremont, R, and Khodakhah, K. It's not just the basal ganglia: cerebellum as a target for dystonia therapeutics. Mov Disord (2017) 32:1537–45. doi:10.1002/mds.27123
31. Bologna, M, and Berardelli, A. Cerebellum: an explanation for dystonia? Cerebellum Ataxias (2017) 4:6. doi:10.1186/s40673-017-0064-8
32. Prudente, CN, Hess, EJ, and Jinnah, HA. Dystonia as a network disorder: what is the role of the cerebellum? Neuroscience (2014) 260:23–35. doi:10.1016/j.neuroscience.2013.11.062
33. Filip, P, Lungu, OV, and Bares, M. Dystonia and the cerebellum: a new field of interest in movement disorders? Clin Neurophysiol (2013) 124:1269–76. doi:10.1016/j.clinph.2013.01.003
34. Sadnicka, A, Hoffland, BS, Bhatia, KP, van de Warrenburg, BP, and Edwards, MJ. The cerebellum in dystonia - help or hindrance? Clin Neurophysiol (2012) 123:65–70. doi:10.1016/j.clinph.2011.04.027
35. LeDoux, MS. Animal models of dystonia: lessons from a mutant rat. Neurobiol Dis (2011) 42:152–61. doi:10.1016/j.nbd.2010.11.006
36. LeDoux, MS, and Lorden, JF. Abnormal cerebellar output in the genetically dystonic rat. Adv Neurol (1998) 78:63–78.
37. Morigaki, R, Miyamoto, R, Matsuda, T, Miyake, K, Yamamoto, N, and Takagi, Y. Dystonia and cerebellum: from bench to bedside. Life (Basel) (2021) 11:776. doi:10.3390/life11080776
38. Fremont, R, Tewari, A, Angueyra, C, and Khodakhah, K. A role for cerebellum in the hereditary dystonia DYT1. Elife (2017) 6:e22775. doi:10.7554/eLife.22775
39. Zhang, L, Yokoi, F, Jin, YH, DeAndrade, MP, Hashimoto, K, Standaert, DG, et al. Altered dendritic morphology of Purkinje cells in Dyt1 ΔGAG knock-in and purkinje cell-specific Dyt1 conditional knockout mice. PLoS One (2011) 6:e18357. doi:10.1371/journal.pone.0018357
40. Song, CH, Bernhard, D, Hess, EJ, and Jinnah, HA. Subtle microstructural changes of the cerebellum in a knock-in mouse model of DYT1 dystonia. Neurobiol Dis (2014) 62:372–80. doi:10.1016/j.nbd.2013.10.003
41. Liu, Y, Xing, H, Wilkes, B, Yokoi, F, Chen, H, Vaillancourt, D, et al. The abnormal firing of Purkinje cells in the knockin mouse model of DYT1 dystonia. Brain Res Bull (2020) 165:14–22. doi:10.1016/j.brainresbull.2020.09.011
42. Voelkl, B, Altman, NS, Forsman, A, Forstmeier, W, Gurevitch, J, Jaric, I, et al. Reproducibility of animal research in light of biological variation. Nat Rev Neurosci (2020) 21:384–93. doi:10.1038/s41583-020-0313-3
43. Liu, Y, Xing, H, Ernst, AF, Liu, C, Maugee, C, Yokoi, F, et al. Hyperactivity of Purkinje cell and motor deficits in C9orf72 knockout mice. Mol Cell Neurosci (2022) 121:103756. doi:10.1016/j.mcn.2022.103756
44. Lyu, S, Xing, H, DeAndrade, MP, Perez, PD, Yokoi, F, Febo, M, et al. The role of BTBD9 in the cerebellum, sleep-like behaviors and the restless legs syndrome. Neuroscience (2020) 440:85–96. doi:10.1016/j.neuroscience.2020.05.021
45. Lyu, S, Xing, H, Liu, Y, Girdhar, P, Yokoi, F, and Li, Y. Further studies on the role of BTBD9 in the cerebellum, sleep-like behaviors and the restless legs syndrome. Neuroscience (2022) 505:78–90. doi:10.1016/j.neuroscience.2022.10.008
46. Yokoi, F, Yang, G, Li, J, DeAndrade, MP, Zhou, T, and Li, Y. Earlier onset of motor deficits in mice with double mutations in Dyt1 and Sgce. J Biochem (2010) 148:459–466.
47. Tian, J, Tep, C, Benedick, A, Saidi, N, Ryu, JC, Kim, ML, et al. p75 regulates Purkinje cell firing by modulating SK channel activity through Rac1. J Biol Chem (2014) 289:31458–72. doi:10.1074/jbc.M114.589937
48. Yokoi, F, Dang, MT, Yang, G, Li, J, Doroodchi, A, Zhou, T, et al. Abnormal nuclear envelope in the cerebellar Purkinje cells and impaired motor learning in DYT11 myoclonus-dystonia mouse models. Behav Brain Res (2012) 227:12–20. doi:10.1016/j.bbr.2011.10.024
49. Yokoi, F, Dang, MT, Zhou, T, and Li, Y. Abnormal nuclear envelopes in the striatum and motor deficits in DYT11 myoclonus-dystonia mouse models. Hum Mol Genet (2012) 21:916–25. doi:10.1093/hmg/ddr528
50. Breakefield, XO, Blood, AJ, Li, Y, Hallett, M, Hanson, PI, and Standaert, DG. The pathophysiological basis of dystonias. Nat Rev Neurosci (2008) 9:222–34. doi:10.1038/nrn2337
51. Gonzalez-Alegre, P. Advances in molecular and cell biology of dystonia: focus on torsinA. Neurobiol Dis (2019) 127:233–41. doi:10.1016/j.nbd.2019.03.007
52. Berryman, D, Barrett, J, Liu, C, Maugee, C, Waldbaum, J, Yi, D, et al. Motor deficit and lack of overt dystonia in Dlx conditional Dyt1 knockout mice. Behav Brain Res (2023) 439:114221. doi:10.1016/j.bbr.2022.114221
53. Yokoi, F, Chen, HX, Oleas, J, Dang, MT, Xing, H, Dexter, KM, et al. Characterization of the direct pathway in Dyt1 ΔGAG heterozygous knock-in mice and dopamine receptor 1-expressing-cell-specific Dyt1 conditional knockout mice. Behav Brain Res (2021) 411:113381. doi:10.1016/j.bbr.2021.113381
54. Yokoi, F, Oleas, J, Xing, H, Liu, Y, Dexter, KM, Misztal, C, et al. Decreased number of striatal cholinergic interneurons and motor deficits in dopamine receptor 2-expressing-cell-specific Dyt1 conditional knockout mice. Neurobiol Dis (2020) 134:104638. doi:10.1016/j.nbd.2019.104638
55. Sciamanna, G, Hollis, R, Ball, C, Martella, G, Tassone, A, Marshall, A, et al. Cholinergic dysregulation produced by selective inactivation of the dystonia-associated protein torsinA. Neurobiol Dis (2012) 47:416–27. doi:10.1016/j.nbd.2012.04.015
56. Yokoi, F, Dang, MT, Li, J, Standaert, DG, and Li, Y. Motor deficits and decreased striatal dopamine receptor 2 binding activity in the striatum-specific Dyt1 conditional knockout mice. Plos One (2011) 6:e24539. doi:10.1371/journal.pone.0024539
57. Yokoi, F, Dang, MT, Mitsui, S, Li, J, and Li, Y. Motor deficits and hyperactivity in cerebral cortex-specific Dyt1 conditional knockout mice. J Biochem (2008) 143:39–47. doi:10.1093/jb/mvm191
58. Pappas, SS, Li, J, LeWitt, TM, Kim, JK, Monani, UR, and Dauer, WT. A cell autonomous torsinA requirement for cholinergic neuron survival and motor control. Elife (2018) 7:e36691. doi:10.7554/eLife.36691
59. Wilkes, BJ, DeSimone, JC, Liu, Y, Chu, WT, Coombes, SA, Li, Y, et al. Cell-specific effects of Dyt1 knock-out on sensory processing, network-level connectivity, and motor deficits. Exp Neurol (2021) 343:113783. doi:10.1016/j.expneurol.2021.113783
60. Liu, Y, Xing, H, Sheng, W, Singh, KN, Korkmaz, AG, Comeau, C, et al. Alteration of the cholinergic system and motor deficits in cholinergic neuron-specific Dyt1 knockout mice. Neurobiol Dis (2021) 154:105342. doi:10.1016/j.nbd.2021.105342
61. Yokoi, F, Dang, MT, and Li, Y. Improved motor performance in Dyt1 Delta GAG heterozygous knock-in mice by cerebellar Purkinje-cell specific Dyt1 conditional knocking-out. Behav Brain Res (2012) 230:389–98. doi:10.1016/j.bbr.2012.02.029
62. Bostan, AC, and Strick, PL. The basal ganglia and the cerebellum: nodes in an integrated network. Nat Rev Neurosci (2018) 19:338–50. doi:10.1038/s41583-018-0002-7
63. DeSimone, JC, Pappas, SS, Febo, M, Burciu, RG, Shukla, P, Colon-Perez, LM, et al. Forebrain knock-out of torsinA reduces striatal free-water and impairs whole-brain functional connectivity in a symptomatic mouse model of DYT1 dystonia. Neurobiol Dis (2017) 106:124–32. doi:10.1016/j.nbd.2017.06.015
64. Washburn, S, Oñate, M, Yoshida, J, Vera, J, Bhuvanasundaram, R, Khatami, L, et al. The cerebellum directly modulates the substantia nigra dopaminergic activity. Nat Neurosci (2024) 27:497–513. doi:10.1038/s41593-023-01560-9
Keywords: Purkinje cells, dystonia, torsinA, DYT1, electrophysiology
Citation: Xing H, Girdhar P, Liu Y, Yokoi F, Vaillancourt DE and Li Y (2025) Subtle changes in Purkinje cell firing in Purkinje cell-specific Dyt1 ΔGAG knock-in mice. Dystonia 4:14148. doi: 10.3389/dyst.2025.14148
Received: 02 December 2024; Accepted: 15 January 2025;
Published: 29 January 2025.
Edited by:
Roy Sillitoe, Baylor College of Medicine, United StatesCopyright © 2025 Xing, Girdhar, Liu, Yokoi, Vaillancourt and Li. This is an open-access article distributed under the terms of the Creative Commons Attribution License (CC BY). The use, distribution or reproduction in other forums is permitted, provided the original author(s) and the copyright owner(s) are credited and that the original publication in this journal is cited, in accordance with accepted academic practice. No use, distribution or reproduction is permitted which does not comply with these terms.
*Correspondence: Yuqing Li, eXVxaW5nbGlAdWZsLmVkdQ==