- 1Translational Medicine Program, The Hospital for Sick Children, Toronto, ON, Canada
- 2Department of Laboratory Medicine and Pathobiology, University of Toronto, Toronto, ON, Canada
Obesity, characterised by excessive fat accumulation, is a complex chronic condition that results from dysfunctional adipose tissue expansion due to prolonged calorie surplus. This leads to rapid adipocyte enlargement that exceeds the support capacity of the surrounding neurovascular network, resulting in increased hypoxia, inflammation, and insulin resistance. Intermittent fasting (IF), a dietary regimen that cycles between periods of fasting and eating, has emerged as an effective strategy to combat obesity and improve metabolic homeostasis by promoting healthy adipose tissue remodeling. However, the precise molecular and cellular mechanisms behind the metabolic improvements and remodeling of white adipose tissue (WAT) driven by IF remain elusive. This review aims to summarise and discuss the relationship between IF and adipose tissue remodeling and explore the potential mechanisms through which IF induces alterations in WAT. This includes several key structural changes, including angiogenesis and sympathetic innervation of WAT. We will also discuss the involvement of key signalling pathways, such as PI3K, SIRT, mTOR, and AMPK, which potentially play a crucial role in IF-mediated metabolic adaptations.
Introduction
Obesity is a growing epidemic, impacting individuals and societies on a global scale. It is a complex chronic disease marked by the accumulation of excess body fat, or adiposity, which negatively affects an individual’s health [1]. The impact of obesity extends beyond increased body weight and is linked with a myriad of cardiometabolic diseases, such as type 2 diabetes (T2D), hypertension, and atherosclerosis, in addition to respiratory diseases and certain types of cancer [2, 3]. White adipose tissue (WAT) is a critical endocrine organ implicated in the progression of obesity. WAT is associated with an extensive neurovascular network and contains a heterogeneous population of cells, including mature adipocytes, adipose stem and progenitor cells (ASPCs) and vascular endothelial and immune cells [4]. These interactions contribute to the overall function of WAT in regulating whole-body metabolism and systemic homeostasis.
Adipose tissue remodeling is a biological process that involves changes in the morphology, cellular composition, and function of adipose tissue in response to physiological or pathological stimuli (Figure 1) [6]. A central component of the remodeling process is WAT expansion, which is characterised by two physiological processes: adipocyte hypertrophy, which is the increase in cell size, and hyperplasia, which leads to an increase in adipocyte cell number through adipogenesis [5]. The process of adipogenesis involves the differentiation of ASPCs into mature adipocytes and is controlled by a subset of regulatory signalling pathways [7].
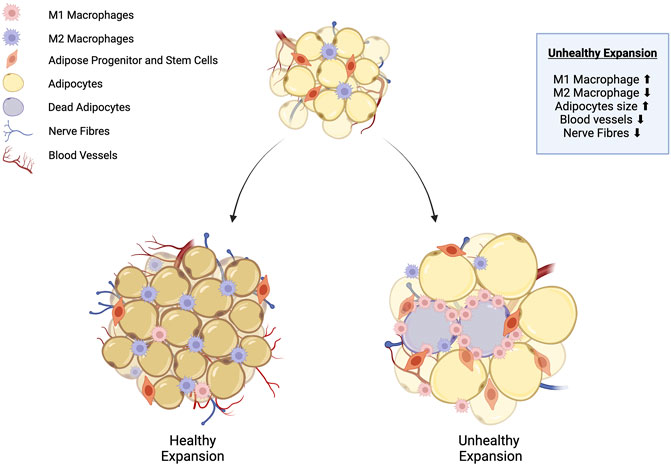
Figure 1. Adipose tissue remodeling: healthy vs. unhealthy WAT expansion. Healthy white adipose tissue (WAT) expansion is characterised by the proliferation of adipose stem and progenitor cells (ASPCs) into numerous small adipocytes. At the same time, anti-inflammatory M2 macrophages are recruited into the adipose tissue, and expansion is supported by the growth of the surrounding neurovascular architecture. Conversely, unhealthy WAT expansion is characterised by impaired differentiation of ASPCs into mature adipocytes. Lipids accumulate within existing adipocytes, causing them to progressively enlarge. Some adipocytes undergo apoptosis due to inadequate nutrient supply from blood vessels, which triggers the recruitment of pro-inflammatory M1 macrophages, leading to increased inflammation. Adapted from Choe et al. [5], licensed under CC BY 4.0. Created with BioRender.com.
It is also accompanied by the development of a supportive neurovascular architecture through signalling mechanisms such as the canonical vascular endothelial growth factor (VEGF)/VEGF Receptor 2 (VEGFR2). VEGF signalling is known to regulate angiogenesis, the growth of blood vessels, sympathetic innervation, and the extension and branching of sympathetic nerve fibres within adipose tissue [8]. Angiogenesis is critical for the delivery of essential nutrients, the removal of metabolic waste, the transport of adipokines, and the mobilisation of free fatty acids released during lipolysis [9]. Sympathetic innervation plays a significant role in the regulation of lipolysis, adipocyte proliferation, and thermogenesis [10–12].
A chronic imbalance between energy intake and expenditure can give rise to dysfunctional adipose tissue expansion, where adipocyte hypertrophy predominates and exceeds the capacity of the surrounding neurovascular network. This imbalance can result in hypoxia, triggering a cascade of inflammatory responses that ultimately contribute to the development of insulin resistance [13–15]. Thus, this highlights the considerable importance of adipose tissue remodeling in obesity, positioning it as a key target for therapeutic intervention.
Current strategies for managing obesity include dietary regimens, physical exercise, pharmacotherapy, and, in certain cases, bariatric surgery [16–19]. Among dietary approaches, calorie restriction (CR), which involves a decrease in total calories consumed, has been extensively studied and clinically implemented. CR is linked to numerous health benefits, including improvements in cardiovascular and metabolic health, cognitive function, and longevity [20–23]. However, maintaining weight loss through long-term adherence to a calorie-restricted regimen can be difficult, often resulting in poor compliance and subsequent weight regain once the regimen is discontinued [24, 25].
Given these challenges, intermittent fasting (IF) has emerged as a promising dietary strategy over the past decade. Unlike CR, it involves cycles of defined fasting and eating periods without necessarily limiting the number of calories an individual consumes. Instead, IF restricts food consumption to certain hours of the day or specific days of the week. This regimen yields metabolic benefits similar to CR, including reduced body mass, improved adipose tissue inflammation and insulin sensitivity, and improved cardiometabolic health without reducing calorie intake [26–31]. Directly comparing the metabolic improvements between CR and IF requires additional studies, which are currently limited and ongoing [32–34].
IF encompasses a diverse range of dietary protocols that vary in the duration, frequency, and extent of calorie reduction during fasting periods. One notable strategy is alternate day fasting (ADF), which can be further categorised as complete ADF, involving zero calorie intake on fasting days, or modified ADF, which allows approximately 25% of an individual’s daily calorie intake to be consumed during fasting periods. This adaptation mitigates some of the challenges associated with ADF and improves sustainability and adherence [35].
Periodic Fasting (PF) represents another form of IF, and it involves extended fasting periods or significantly reduced calorie intake interspersed with normal eating periods. Unlike ADF, PF typically involves longer fasting intervals of 2 days or more [29, 36]. The 5:2 IF regimen, one of the most recognised and popularised, allows unrestricted eating 5 days a week, with reduced calorie consumption on either two consecutive or non-consecutive days [37].
Time-restricted feeding (TRF) is a protocol that restricts food intake to a specific 8-h daily window, outside of which only water is allowed [38]. Recent clinical studies have highlighted that early TRF, with the window starting at 06:00 AM, further improves metabolic parameters such as fasting glucose and insulin sensitivity [39, 40]. These benefits are mediated, in part, by the synchronicity of TRF with our circadian rhythm. Ramadan fasting (RF) is a unique form of TRF practised by Muslims during the month of Ramadan, which involves restricted eating and drinking from dawn (Suhur) to sunset (Iftar) [41]. The duration of fasting depends on the time of year and geographical location. A recent meta-analysis by Fernando et al highlighted reductions in body weight and fat mass following RF, along with improvements in several metabolic markers such as fasting glucose and low-density lipoprotein levels [42, 43].
Despite the growing popularity and adoption of various IF protocols, the underlying biological mechanisms that facilitate these benefits remain to be elucidated. Existing research has demonstrated that IF promotes a range of metabolic adaptations, including enhanced lipolysis, β-oxidation, gut microbiota changes, and increased autophagy [44–48]. IF also significantly influences the browning of WAT, leading to the formation of beige adipocytes, which contain smaller lipid droplets and a higher mitochondrial density [26, 28, 49, 50]. This browning effect facilitates heat dissipation through respiratory uncoupling via uncoupling protein 1 (UCP1), a process known as WAT thermogenesis, which is critical in regulating energy expenditure and metabolic homeostasis [26, 28, 49, 50]. These processes contribute to the metabolic benefits observed with IF, including improved insulin sensitivity and glucose tolerance.
However, significant gaps persist in our understanding of the specific molecular pathways involved and the differential effects of IF on various tissues and organs. In this review, we aim to explore the signalling pathways, with a focus on adipose tissue that is influenced by IF and its downstream effects on adipose tissue remodeling. We also aim to identify key areas for future research to improve our current knowledge of the molecular targets that drive these metabolic adaptations. This understanding will set the stage for the development of pharmacological interventions that can mimic the beneficial effects of IF, potentially offering new strategies for the management of obesity.
Intermittent fasting and associated signalling pathways
Phosphatidylinositol 3-kinase (PI3K) and protein kinase B (Akt) pathways
The PI3K/Akt signalling pathway coordinates various anabolic processes that are essential for maintaining cell growth and proliferation, glucose and lipid metabolism, and autophagy. This pathway is crucial for insulin signal transduction and exerts beneficial effects on glucose homeostasis through the regulation of FOXO1 and GSK3β, mitochondrial biogenesis via mTORC1, and lipogenesis through the regulation of PPARγ and SREBP1-c [50].
Activation of PI3K/Akt signalling in adipocytes has been linked to improved insulin sensitivity and glucose tolerance, while also promoting lipogenesis over lipolysis in vitro and in vivo [51–54]. During the refeeding phase of IF in mice, there is an upregulation of CDC-like kinase 2 (CLK2) in brown adipose tissue, which contributes to the increased energy expenditure observed with IF. This upregulation may be regulated by insulin and PI3K signalling since treatment with a PI3K inhibitor has been shown to ameliorate the upregulation of CLK2 [55].
Although direct evidence connecting IF with PI3K/Akt signalling in WAT is still lacking, CR in mice has been shown to improve insulin sensitivity and promote lipid metabolism through Akt activation, particularly in the liver [56]. Catalpol, a naturally derived drug, has been shown to alleviate hepatic insulin resistance and improve glucose homeostasis in mice by stimulating AMP-activated protein kinase (AMPK) and PI3K/Akt signalling. Knockdown of AMPK in HepG2 cells was observed to prevent Akt phosphorylation and activation induced by Catalpol [57].
Furthermore, in a mouse model of diet-induced obesity (DIO), a 5-week ADF regimen mitigated obesity-induced remodeling of the atria. It also showed significant improvements in glucose tolerance and insulin sensitivity via SIRT3 and its downstream activation of AMPK and Akt [58]. Additionally, by modelling chronic myocardial ischaemia in rats, Katare et al demonstrated that long-term IF led to a substantial improvement in survival rates through activation of the BDNF/VEGF/PI3K signalling cascade in the heart, with a significant upregulation of phospho-Akt [59].
Conversely, the downregulation of PI3K/Akt signalling is also highlighted in specific circumstances. Butein, a phytochemical, can induce WAT beiging in DIO mice through inhibition of PI3K and downstream Akt signalling, leading to activation of PRDM4, a regulator of energy expenditure and thermogenesis [60]. A study of ADF in rats showed improvement in age-associated hypertrophy via downregulation of PI3K/Akt signalling in the heart’s left ventricle [61]. Moreover, PI3K/Akt activation was also inhibited in the hippocampal region following IF, activating GSK3β and promoting neuronal differentiation in a mouse model of Alzheimer’s disease [62]. Similarly, the hypothalamus showed reduced activation of PI3K/Akt/mTOR signalling following short-term fasting in rats [63].
IF facilitates the metabolic switch between catabolic and anabolic states in response to fasting and refeeding. This may result in the inhibition or activation of PI3K/Akt signalling. These findings illustrate the complex and context-dependent relationship between IF and PI3K/Akt signalling in various tissues, including adipose tissue, liver, heart, and brain. This dual regulation may explain how IF promotes insulin sensitivity while also stimulating catabolic processes such as WAT lipolysis and thermogenesis. Further research is essential to unravel the precise mechanisms of IF-induced modulation of the PI3K/Akt pathway in WAT.
Sirtuins (SIRTs) pathway
SIRTs are a family of nicotine adenine dinucleotide (NAD)+-dependent deacetylases that regulate numerous cellular processes, including metabolism, ageing, and oxidative stress [64, 65]. SIRTs catalyse the removal of acyl groups from target proteins and function as metabolic regulators in response to changes in NAD+ levels. The SIRT family consists of seven members, SIRT1 through SIRT7, each residing in specific subcellular compartments [64].
In individuals with obesity, SIRT6 expression in WAT was found to be significantly reduced [66]. Mice lacking SIRT6 in adipocytes, when subjected to a high-fat diet (HFD), manifested exacerbated insulin resistance and inflammation [66]. Similarly, adipocyte-specific SIRT6 knockout mice undergoing IF failed to show improvements in glucose homeostasis and insulin sensitivity. The absence of SIRT6 also led to reduced adipose browning and lower energy expenditure, suggesting that SIRT6 is a critical mediator of the metabolic improvements induced by IF [67].
Moreover, CR in rats and fasting in humans have been shown to upregulate SIRT1 expression in WAT [68, 69]. SIRT1 promotes WAT browning and increases energy expenditure by deacetylating PPARγ [70]. However, Boutant et al revealed that while SIRT1 overexpression leads to similar improvements in insulin sensitivity, it cannot replicate the effects of ADF on WAT metabolism, including increased mitochondrial respiration and distinct transcriptional alterations in epididymal WAT [71].
SIRT7 can also significantly affect lipid metabolism and thermogenesis [72, 73]. Yoshizawa et al found that whole-body and brown adipose tissue-specific SIRT7 knockout mice display augmented body temperature and energy expenditure along with increased UCP1 expression [73]. Furthermore, Tang et al demonstrated that IF can promote SIRT7 stability by regulating AMPK activity, thereby activating the GSK3β-SIRT7 axis in the context of enhancing the anti-tumour effects of chemotherapy [74]. These findings underscore the potential role of SIRTs in WAT metabolism and remodeling. Nevertheless, additional research is required to delineate the contributions of SIRT7 and other SIRTs within WAT.
Mammalian target of the rapamycin (mTOR) pathway
The mTOR signalling pathway is a critical regulator of autophagy, among its numerous roles in influencing growth, proliferation, glucose, and lipid metabolism [75]. Two functional protein complexes are involved in the regulation of mTOR signalling: mTOR complex 1 (mTORC1) and mTOR complex 2 (mTORC2). mTORC1 is known to directly phosphorylate and inhibit the activity of Unc-51-like kinase 1 (ULK1), thus negatively regulating autophagy [75].
The mTOR pathway is activated under conditions of excess calorie intake. This activation facilitates de novo lipogenesis by activating sterol regulatory element-binding protein 1 (SREBP1) while concurrently suppressing lipolysis by downregulating the expression of lipolytic enzymes such as adipose triglyceride lipase (ATGL) and hormone-sensitive lipase (HSL) [76, 77]. Moreover, activation of mTORC1 is associated with increased adipogenesis, which supports the storage of excess lipids [78]. Knock out of raptor, a component of mTORC1, in adipocytes results in increased insulin sensitivity, reduced fat mass, and elevated energy expenditure in mice. This is achieved through the beiging of WAT, as evidenced by the upregulation of UCP1 and other browning markers [79]. These observed metabolic benefits are similar to those promoted by IF, highlighting the role of mTOR signalling in the regulation of WAT function and metabolism.
The inhibitory effects of IF on mTOR signalling in WAT have been largely unexplored. However, previous studies have shown the beneficial effects of IF and mTOR signalling in the heart. This is mediated through the intermittent activation of the transcription factor EB (TFEB), a positive regulator of autophagy [80]. Ma et al showed that IF can reduce the activation of mTOR, a negative regulator of TFEB, thereby stimulating TFEB and facilitating protective autophagic effects in a mouse model of desmin-related cardiomyopathy [81–83]. Notably, the phytochemical Acteoside can stimulate beige adipocyte formation in vitro via the mTORC1-TFEB signalling pathway, where upregulation of browning markers such as PGC-1α and UCP1 has been observed [84]. Whether IF can mediate autophagy and other positive effects on WAT remodeling via mTOR-TFEB signalling remains unknown and warrants further investigation.
AMPK as the upstream regulator of PI3K/Akt, SIRTs, and mTOR signalling
AMPK is a widely expressed serine/threonine kinase that functions as a metabolic switch, responding to changes in adenosine monophosphate (AMP)/adenosine triphosphate (ATP) levels within the cell. AMPK functions to restore energy balance by favouring catabolic processes that generate ATP while suppressing anabolic pathways [85]. AMPK is activated in response to various metabolic challenges, including exercise, cold exposure, and fasting [86–88]. Fasting can activate AMPK directly or indirectly, through the increased production of metabolic hormones such as catecholamines and adiponectin [86, 89, 90].
AMPK can activate PI3K/Akt signalling as demonstrated in vitro by treating differentiated 3T3-L1 adipocytes with AICAR, an AMPK agonist [91]. Impairments in AMPK and PI3K/Akt signalling, observed in obesity and T2D, may contribute to insulin resistance in humans [92, 93]. However, the relationship between AMPK and PI3K/Akt is complex and context-dependent, as activation of AMPK favours catabolic processes. Fasting-induced activation of AMPK can, in contrast, directly inhibit mTOR, leading to increased autophagy via ULK1 activation. This process also promotes catabolic pathways such as lipolysis and β-oxidation while suppressing adipogenesis and lipogenesis [94].
IF triggers significant fluctuations in cellular energy levels, providing a repeated stimulus that may give rise to the remodeling of WAT via activation of AMPK. Several studies have highlighted AMPK as a critical factor in regulating glucose and lipid metabolism, and driving thermogenesis via WAT browning [90, 95, 96]. Conversely, impairment in AMPK activity is associated with various metabolic disorders, including obesity and insulin resistance, type 2 diabetes and fatty liver disease [88, 90]. Although IF has recently been demonstrated to promote AMPK activation and remodeling in the hearts of rats, its role in facilitating IF-induced remodeling of WAT remains elusive [97].
Intermittent fasting and angiogenic remodeling
IF, similar to cold exposure and exercise, is a potent stimulus for promoting adipose tissue thermogenesis via the beiging of WAT [26, 49]. This is favourable in the context of obesity, where increasing energy expenditure may be one non-pharmacological approach to its management. IF is known to induce brown fat-like changes via adipose angiogenic remodeling. 2:1 IF (48-hour feeding, 24-hour fasting) under high-fat diet (HFD) conditions resulted in improved glucose tolerance, insulin sensitivity, and increased energy expenditure. These metabolic benefits are dependent on an IF-stimulated increase in VEGF-A expression, which leads to visceral WAT browning (upregulation of Adrb3, Ppargc1a, Cidea, Ucp1) via M2-like macrophage polarisation (upregulation of Clec10a, Il10, Ym1) [26]. IF can mediate browning in perigonadal and inguinal WAT (pWAT and iWAT, respectively), and higher energy expenditure was observed under both normal-chow and HFD-IF regimens [28].
The AMPK-SIRT1-PGC1α signalling axis may be a pivotal driver of VEGF-A-mediated angiogenic remodeling and browning in WAT in response to IF (Figure 2). This pathway’s relevance was illustrated in HepG2 human hepatoma cells, where AICAR-stimulated AMPK activation resulted in increased VEGF-A production [98]. Glucose starvation for 4 h in HepG2 cells, in vitro conditions that mimic fasting, resulted in elevated phospho-AMPK and VEGF-A mRNA expression. Furthermore, pharmacological activation of SIRT1 led to significant metabolic improvements in DIO mice, along with improved vasculature and reduced fibrosis. In 3T3-L1 preadipocytes, SIRT1 activation was associated with the increased expression of angiogenic factors, including VEGF-A [99]. This provides evidence for SIRT1 being an upstream regulator of VEGF-A. Activation of AMPK is capable of phosphorylating and stimulating PGC-1α in skeletal muscle and WAT, an essential regulator of mitochondrial biogenesis and metabolism [100, 101]. PGC-1α activation is critical for VEGF-mediated angiogenesis in skeletal muscle, although its causative role in IF-induced WAT angiogenesis has yet to be established [102]. Nonetheless, Ppargc1a was significantly upregulated in WAT following IF in mice and may, therefore, be an upstream regulator of WAT angiogenesis [26]. The AMPK-SIRT1- PGC1α signalling axis was, in fact, directly activated by the anti-diabetic drug Canagliflozin in adipocytes in vitro, with downstream effects including increased thermogenesis and energy expenditure [103]. This provides further support for the likely involvement of this pathway in IF. Although direct evidence linking IF to the activation of AMPK, SIRT1, and PGC-1α in WAT has yet to be shown, CR can activate this pathway in the heart and mitigate myocardial injury following reperfusion [104].
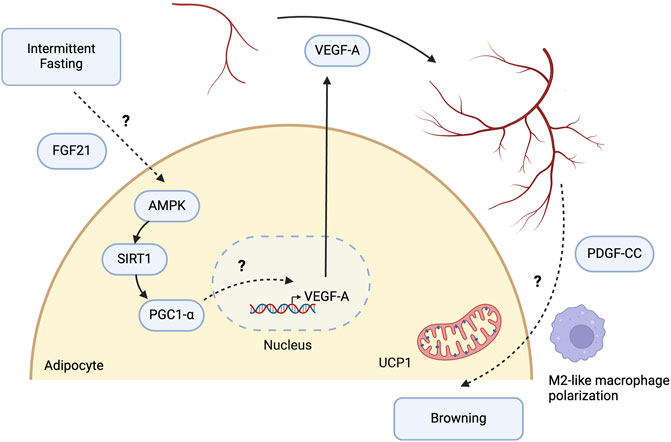
Figure 2. Potential molecular pathways involved in intermittent fasting-induced angiogenic remodeling of white adipose tissue. Intermittent fasting (IF) induces the expression of vascular endothelial growth factor A (VEGF-A) in white adipose tissue (WAT), promoting angiogenesis and browning. Liver-derived fibroblast growth factor 21 (FGF21) is indispensable for IF-mediated angiogenesis and browning in WAT. FGF21 upregulates the AMPK-SIRT1-PGC1-α signalling pathway and increases energy expenditure in adipocytes, highlighting FGF21 as one of the intermediary mechanisms between IF and AMPK activation. While PGC1-α regulates VEGF-A-mediated angiogenesis in skeletal muscle, its role in WAT remains to be determined. The mechanistic link between increased angiogenesis and WAT browning may involve endothelial cells releasing platelet-derived growth factor CC (PDGF-CC) in response to VEGF-A, leading to WAT browning. It may also be driven by alterations in immune cells, such as polarisation towards M2-like macrophages. However, the specific mechanisms remain to be elucidated. Created with BioRender.com.
Fibroblast growth factor 21 (FGF21) also plays a crucial role in regulating VEGF-A expression. In mice with liver-specific knockout of FGF21 subjected to IF for 16 weeks, there was a lack of angiogenic growth and browning in WAT, as indicated by the reduced expression of browning markers such as Ucp1, Ppargc1a, and Elovl6 [105]. Additionally, IF-mediated anti-inflammatory effects were lost following FGF21 knockout, as evidenced by an increase in pro-inflammatory M1-like macrophage markers. Since M2-like polarisation is essential for IF-induced browning, liver-derived FGF21 may serve as a critical upstream regulator in this process. Interestingly, Abu-Odeh et al highlighted that browning of iWAT following treatment with the β-adrenergic agonist CL-316,243, which mimics cold exposure, still occurred despite the liver-specific knockout of FGF21. These findings suggest that hepatic and adipocyte-derived FGF21 may have differing effects under various physiological stimuli, with liver-derived FGF21 being crucial for IF-induced browning and anti-inflammation, while adipocyte-derived FGF21 plays a key role in β-adrenergic receptor-mediated thermogenesis [106]. Nevertheless, Chau et al established that FGF21 increases energy expenditure through the phosphorylation and activation of AMPK, which in turn activates SIRT1 and PGC-1α both in vitro and in vivo [107]. Thus, IF may induce VEGF-A expression and angiogenic remodeling via an upstream pathway involving FGF21, AMPK, SIRT1, and PGC-1α. Further research is needed to confirm these mechanisms and to fully elucidate the role of FGF21 in IF-mediated angiogenesis.
The crosstalk between white adipocytes and endothelial cells is vital for angiogenesis and WAT browning. Seki et al investigated the effects of adrenergic activation induced by cold exposure and CL-316,243 treatment, which elicited a browning response similar to that observed with IF. Their research identified platelet-derived growth factor CC (PDGF-CC) as an endothelial-derived soluble factor activated by VEGF-A that promotes the differentiation of adipocyte progenitor cells towards a beige phenotype [108]. Furthermore, Seki et al demonstrated that deletion of VEGF receptor 1 (VEGFR1) in endothelial cells, a receptor that typically functions as a sink for VEGF-A and thus inhibits its function, results in WAT browning [109]. VEGF-A likely interacts with other receptors on endothelial cells besides VEGFR1, including VEGFR2 and neuropilin-2, indicating a complex network of potential signalling interactions between adipocytes and endothelial cells [110].
CR elicits a type 2 immune response similar to IF, mediating the browning of WAT [20]. Under CR conditions, SIRT1 levels were elevated in macrophages and eosinophils, a response likely driven by IL-4 [111]. In a contrasting study, J. Park et al transplanted adipose tissue overexpressing VEGF-A into DIO mice, offering a direct parallel to the IF-induced elevation of VEGF-A described by Kim et al [26, 112]. Following transplantation, angiogenic remodeling and the development of beige adipocytes were observed, independent of IL-4 [112]. Group 2 innate lymphoid cells (ILC2) have been extensively researched in adipose tissue for their role in fostering a type 2 inflammatory environment. The essential cytokines, IL-5 and IL-13 are critical for the activation of eosinophils and M2-like macrophages, thereby influencing WAT beiging and thermogenesis [113–115]. Given that VEGF-A does not directly induce the M2-like polarisation of macrophages necessary for the browning effects seen with IF, the interplay between endothelial cells, macrophages, and other immune cells, as well as proangiogenic factors will be important in determining the mechanism underlying IF-induced WAT browning.
Intermittent fasting and sympathetic innervation
Sympathetic innervation is a key regulator of lipolysis, allowing fat mobilisation from adipose tissue in response to the body’s energy demands. In response to physiological stimuli, activation of the SNS drives the release of norepinephrine (NE) from local sympathetic nerves into the adipocyte microenvironment. This subsequently activates β-adrenergic G-protein coupled receptors expressed on adipocytes, including ADRB1, ADRB2, and ADRB3, resulting in the decoupling of the GS protein and activation of adenylate cyclase (AC). This results in an increase in levels of intracellular cAMP, which activates protein kinase A (PKA) and culminates in a signalling cascade that phosphorylates key lipolytic enzymes such as HSL and perilipin A (PLIN1a) [116]. Activated HSL translocates to the lipid droplet monolayer, and ATGL is subsequently activated by the release of ABHD5 from activated PLIN1a, catalysing the hydrolysis of triglycerides [117].
Early evidence from Migliorini et al indicated increased sympathetic activity in epididymal WAT after 48 h of fasting, as evidenced by an increased NE turnover rate [118]. Additionally, 48-hour fasting has also been shown to stimulate sympathetic innervation, as evidenced by increased tyrosine hydroxylase (TH) protein content, in both epididymal and retroperitoneal WAT [119]. β-adrenergic activity can alter the expression of GLUT4 in WAT in response to short-term fasting and refeeding, thereby regulating glucose homeostasis [120]. It remains unclear whether IF utilises a similar mechanism to improve glucose homeostasis. IF also increases lipolysis through elevated phosphorylation of HSL and extracellular signal-regulated kinase (ERK), a kinase that activates lipolytic enzymes, including HSL [44]. The upregulation of Adrb3 expression in mouse WAT under IF suggests that sympathetic activation of adrenergic receptors may initiate the downstream lipolytic cascade [26]. A similar mechanism is observed in humans, where propranolol-stimulated blockade of β-adrenergic receptors can mitigate fasting-induced lipolysis [121].
In contrast, Li et al elucidated an opposite effect of IF leading to reduced Adrb3 expression in both BAT and WAT. Their study highlighted the importance of gut microbiota changes in driving WAT browning after every-other-day feeding (EODF), a regimen synonymous with ADF [49]. A separate study using proteomic analysis on fat pads from EODF-treated mice revealed a reduction in ADRB3 and lipolytic pathways, including reduced monoacylglycerol lipase, along with an upregulation in fatty acid synthesis pathways involving enzymes such as ATP-citrate lyase. This suggests a potential mechanism for energy conservation [122]. Given the evident reduction in calorie intake associated with EODF, the observed attenuation of lipolysis may be a metabolic adaptation compared to the isocaloric nature of the 2:1 IF regimen, which activates ADRB3 [26]. These findings underscore the importance of the specific type of IF intervention on WAT remodeling and its downstream functional effects.
The molecular mechanisms by which IF results in adaptive remodeling of the neural architecture of WAT, thereby promoting lipolysis and thermogenesis, remain to be investigated (Figure 3). Current evidence suggests that IF facilitates angiogenesis via upregulation of VEGF-A [26]. Separately, Zhao et al showed that VEGF-A overexpression in transgenic mice not only increases angiogenesis but also promotes sympathetic nerve growth [123]. VEGF-A overexpression leads to downstream activation of ADRB3 and phosphorylation of HSL, as well as increased expression of browning markers including UCP1 and PGC1- α. This highlights a potential mechanism by which IF may mediate lipolysis and browning via VEGF-A-induced sympathetic nerve growth [123].
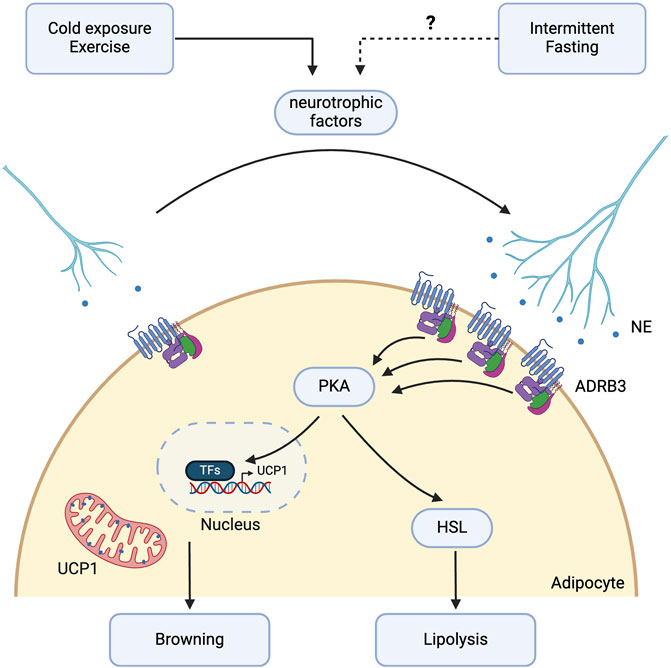
Figure 3. Potential molecular pathways involved in intermittent fasting-induced sympathetic innervation. Intermittent fasting (IF) may promote sympathetic activation in white adipose tissue (WAT) through the β-3 adrenergic signalling pathway. Although direct evidence showing IF’s effect on sympathetic nerve growth in WAT is currently lacking, existing studies suggest that IF may promote this growth through VEGF-A or other neurotrophic factors such as neuronal growth regulator 1 (NEGR1), neurotrophin-3 (NT-3), neuregulin 4 (NRG4), nerve growth factor (NGF), and slit guidance ligand 3 (SLIT3), which are implicated in exercise or cold-induced sympathetic nerve growth. IF enhances β-3 adrenergic receptor expression and downstream lipolysis through activation of hormone-sensitive lipase (HSL). Sympathetic innervation further promotes the browning of WAT via the protein kinase A (PKA) intracellular pathway, increasing the expression of thermogenic genes such as uncoupling protein 1 (UCP1). Created with BioRender.com.
Stimuli, such as exercise and cold exposure, which activate ADRB3 and confer metabolic benefits similar to those of IF, have been observed to stimulate both sympathetic innervation and angiogenesis in WAT. In addition to VEGF-A, several neurotrophic factors released in WAT regulate this remodeling process. Neuronal growth regulator 1 (NEGR1), a cell adhesion molecule that modulates neural innervation in the brain, is significantly associated with body mass index (BMI) in meta-analyses of genome-wide association studies [124, 125]. Exercise training for 10 weeks in C57BL/6 mice induced NEGR1 expression specifically from mature white and beige adipocytes in iWAT, leading to increased neurite growth. The increase in NEGR1 expression has also been observed in humans following treadmill training, with PRDM16, regulated by PPARγ, identified as the critical transcription factor governing NEGR1 expression [126].
Neurotrophin-3 (NT-3) and neuregulin 4 (NRG4) are adipocyte-derived growth factors that mediate sympathetic nerve growth in WAT and promote beige fat formation under cold conditions [8, 10]. NT-3 binds to TrKC receptors in the sympathetic ganglia, while NRG4 functions through ErbB4 [127]. NRG4 can also directly stimulate browning in adipocytes in vitro [128]. Cold-induced production of nerve growth factor (NGF) by adipose eosinophils also stimulates neurite growth [129]. NGF, recognised as an adipokine, targets TrkA receptors in iWAT and is necessary for cold-stimulated beiging [130]. Increased NGF production is attributed to an increased accumulation of adipose eosinophils, driven by increased IL-5 secretion from ILC2s, which in turn is stimulated by IL-33 release from stromal cells in response to cold. Cold-induced sympathetic nerve growth is also mediated by the secretion of slit guidance ligand 3 (SLIT3) by M2-like macrophages, which bind to roundabout guidance receptor 1 (ROBO1) receptors on nerve fibres. The SLIT3-ROBO1 interaction promotes increased NE release, leading to the upregulation of the lipolytic and thermogenic pathways, marked by elevated PKA activity, phosphorylation of HSL, and increased UCP1 expression [131].
A plethora of neurotrophic factors regulate sympathetic nerve growth in WAT in response to exercise and cold exposure. However, whether these factors play a specific role in mediating IF-stimulated adipose tissue remodeling remains to be elucidated. IF is known to shift the immune cell landscape towards a type 2 inflammatory response with increased M2-like macrophage polarisation and eosinophils, as observed in aged mice subjected to IF [132]. This contrasts with the pro-inflammatory environment in obese adipose tissue, characterised by reduced ILC2 and eosinophil populations and a shift towards M1-like macrophages [133–135]. These immune alterations may contribute to the underlying mechanisms leading to reduced sympathetic innervation of WAT in obesity [136]. Furthermore, whether AMPK plays a role in regulating SNS activation remains uncertain. Nevertheless, AMPK is known to directly regulate lipolysis through the phosphorylation of ATGL and HSL [137]. Additionally, the process of lipolysis itself leads to an increase in the AMP:ATP ratio, which may result in the activation of AMPK [138].
Discussion
Recent studies have established IF as an effective, economical, and practical strategy for weight management. Meta-analyses and umbrella reviews conducted in recent years highlight the efficacy of IF as a dietary intervention in improving health outcomes in obese or overweight participants, including reduced body weight and fat mass, favourable lipid profiles, reduced inflammatory markers, and improved fasting insulin as well as plasma glucose levels [139–143]. Beyond its efficacy in obese adult populations, IF may reduce BMI and the risk of cardiovascular disease in adolescents with obesity [144]. Despite the known metabolic health advantages associated with IF, compliance with a dietary regimen like IF can pose a challenge in the long term. However, adherence and feasibility can be maintained by implementing a personalised dietary regimen under the guidance of a professional dietitian and by building flexibility into the programme, allowing users to choose specific days of the week to fast or restrict calories [144].
To mediate the beneficial effects of IF on WAT remodeling and systemic metabolic homeostasis, IF may modulate a complex regulatory network involving signalling pathways such as PI3K/Akt, SIRTs, and mTOR. As AMPK is positioned as the central metabolic switch regulating the downstream pathways, activation of AMPK in response to IF may be integral in promoting a range of cellular processes, including lipolysis, β-oxidation, autophagy, and the browning of WAT. Nevertheless, both gain-of-function and loss-of-function studies of these intracellular pathways, especially in WAT, are necessary to validate their relevance in IF-mediated WAT remodeling. VEGF-A drives IF-induced angiogenesis in WAT, which may, in part, be regulated by the AMPK-SIRT1-PGC1α signalling axis. FGF21 has also emerged as an essential signalling factor upregulated by IF, which can activate AMPK and downstream PGC-1α [105]. VEGF-A, along with other neurotrophic factors, can promote sympathetic nerve growth, highlighting the close interplay observed in the remodeling of the neurovascular architecture of WAT. While IF can promote angiogenesis and sympathetic activation [26], its direct effect on sympathetic nerve growth has not been directly elucidated, with current evidence derived from studies on exercise [126] and cold-induced sympathetic nerve growth [8, 10, 130, 131] in WAT.
Additionally, the current mechanistic understanding of IF remains limited, particularly regarding its direct activation of pathways such as AMPK, PI3K/Akt, various SIRTs, and mTOR in WAT. The majority of the available evidence comes from studies in CR or from other tissues such as the heart [58, 59, 61, 80, 81, 83, 97], liver [56, 57], and brain [62, 63]. Furthermore, the variability between different IF regimens [26, 28, 49, 122] necessitates further investigation into their physiological effects and underlying mechanisms, which is crucial for standardising IF protocols for research and clinical applications. These differences also underscore the importance of thoroughly investigating both the benefits and downsides of IF. For example, ADF has been shown to exacerbate atherosclerosis in mice predisposed to the condition, and TRF increases hepatic insulin resistance in young rats with diet-induced obesity [145, 146]. Understanding these differences and potential adverse effects is essential for identifying appropriate target populations for IF.
It is also essential to consider the cyclical nature of IF with its fasting and refeeding cycles. The periodic activation and inhibition of these signalling pathways may be important in the development and administration of pharmacological mimetics. Intermittent administration of rapamycin can prolong the life of female C57BL/6 mice while negating any metabolic side effects such as impaired insulin sensitivity [147]. Similarly, periodic administration of metformin, an AMPK activator, showed metabolic improvements and weight loss without affecting the mortality of aged mice, despite its toxic side effects at the dosage used [148].
AMPK activators have demonstrated potential for promoting weight loss through the activation of AMPK, leading to the inhibition of fat synthesis and promotion of fat oxidation pathways. However, their clinical use has been predominantly focused on diabetes management rather than weight loss, possibly due to the wide range of targets affected by AMPK activators like metformin [149, 150]. Further research is needed to determine their specific mechanisms of action. The activity of AMPK is also highly dependent on several factors, such as intracellular ATP levels, dosage, and route of administration [151–153]. Additional studies are required to investigate whether AMPK’s functional role in fat metabolism can be separated from its role in glucose regulation, thus supporting its potential as a standalone target for weight loss [154]. Several side effects associated with the use of AMPK activators, including gastrointestinal discomfort, headaches, and fatigue, may compromise adherence, effectiveness, and patient quality of life [153, 155].
In conclusion, IF holds great promise as a dietary intervention for weight loss and the improvement of metabolic health through its multifaceted effects on WAT remodeling. Addressing the gaps in our understanding of the molecular mechanisms driving these processes will enable the development of more targeted drug therapies that can replicate or even enhance the metabolic benefits of IF, potentially circumventing the need for significant dietary changes. Future studies should focus on standardising IF protocols, investigating the interplay between key signalling pathways, and exploring the potential of pharmacological mimetics or a combination of IF and pharmacotherapy to provide a comprehensive approach to obesity management.
Author contributions
NV, QZ, and H-KS conceived and designed the research. All authors contributed to the article and approved the submitted version.
Funding
The authors declare that financial support was received for the research, authorship, and/or publication of this article. H-KS is supported by grants from Canadian Institute of Health Research (CIHR, PJT-162083, PJT-190016), Natural Sciences and Engineering Research Council (NSERC, RGPIN-2016-06610) of Canada, Diabetes Canada (OG-3-23-5715-HS), Canada Foundation for Innovation (CFI, #40249), and Sun Life Financial New Investigator Award of Banting & Best Diabetes Centre (BBDC) of the University of Toronto. NV is supported by Novo-Nordisk Studentship from Banting and Best Diabetes Centre (BBDC) of the University of Toronto and Restracomp Master’s Scholarship from The Hospital for Sick Children. QZ is supported by Doctoral Program from Chinese Scholarship Council (CSC202008340062).
Acknowledgments
We thank Dr. Jacques Togo and Dr. Bruno Rodrigues de Oliveira for their helpful suggestions during the writing of this review.
Conflict of interest
The authors declare that the research was conducted in the absence of any commercial or financial relationships that could be construed as a potential conflict of interest.
References
1. Wharton, S, Lau, DCW, Vallis, M, Sharma, AM, Biertho, L, Campbell-Scherer, D, et al. Obesity in adults: a clinical practice guideline. Can Med Assoc J (2020) 192(31):E875–91. doi:10.1503/cmaj.191707
2. Powell-Wiley, TM, Poirier, P, Burke, LE, Després, JP, Gordon-Larsen, P, and Lavie, CJ. Obesity and cardiovascular disease: a scientific statement from the American heart association. Circulation (2021) 143(21):e984–1010. doi:10.1161/cir.0000000000000973
3. Haslam, DW, and James, WPT. Obesity. The Lancet (2005) 366(9492):1197–209. doi:10.1016/s0140-6736(05)67483-1
4. Emont, MP, Jacobs, C, Essene, AL, Pant, D, Tenen, D, Colleluori, G, et al. A single-cell atlas of human and mouse white adipose tissue. Nature (2022) 603(7903):926–33. doi:10.1038/s41586-022-04518-2
5. Choe, SS, Huh, JY, Hwang, IJ, Kim, JI, and Kim, JB. Adipose tissue remodeling: its role in energy metabolism and metabolic disorders. Front Endocrinol (2016) 7:30. doi:10.3389/fendo.2016.00030
6. Sun, K, Kusminski, CM, and Scherer, PE. Adipose tissue remodeling and obesity. J Clin Invest (2011) 121(6):2094–101. doi:10.1172/jci45887
7. Jeffery, E, Church, CD, Holtrup, B, Colman, L, and Rodeheffer, MS. Rapid depot-specific activation of adipocyte precursor cells at the onset of obesity. Nat Cel Biol (2015) 17(4):376–85. doi:10.1038/ncb3122
8. Pellegrinelli, V, Peirce, VJ, Howard, L, Virtue, S, Türei, D, Senzacqua, M, et al. Adipocyte-secreted BMP8b mediates adrenergic-induced remodeling of the neuro-vascular network in adipose tissue. Nat Commun (2018) 9(1):4974. doi:10.1038/s41467-018-07453-x
9. Cao, Y. Angiogenesis and vascular functions in modulation of obesity, adipose metabolism, and insulin sensitivity. Cel Metab (2013) 18(4):478–89. doi:10.1016/j.cmet.2013.08.008
10. Cui, X, Jing, J, Wu, R, Cao, Q, Li, F, Li, K, et al. Adipose tissue-derived neurotrophic factor 3 regulates sympathetic innervation and thermogenesis in adipose tissue. Nat Commun (2021) 12(1):5362. doi:10.1038/s41467-021-25766-2
11. Foster, MT, and Bartness, TJ. Sympathetic but not sensory denervation stimulates white adipocyte proliferation. Am J Physiology-Regulatory, Integr Comp Physiol (2006) 291(6):R1630–7. doi:10.1152/ajpregu.00197.2006
12. Zeng, W, Pirzgalska, RM, Pereira, MMA, Kubasova, N, Barateiro, A, Seixas, E, et al. Sympathetic neuro-adipose connections mediate leptin-driven lipolysis. Cell (2015) 163(1):84–94. doi:10.1016/j.cell.2015.08.055
13. Hosogai, N, Fukuhara, A, Oshima, K, Miyata, Y, Tanaka, S, Segawa, K, et al. Adipose tissue hypoxia in obesity and its impact on adipocytokine dysregulation. Diabetes (2007) 56(4):901–11. doi:10.2337/db06-0911
14. Xu, H, Barnes, GT, Yang, Q, Tan, G, Yang, D, Chou, CJ, et al. Chronic inflammation in fat plays a crucial role in the development of obesity-related insulin resistance. J Clin Invest (2003) 112(12):1821–30. doi:10.1172/jci200319451
15. Ye, J, Gao, Z, Yin, J, and He, Q. Hypoxia is a potential risk factor for chronic inflammation and adiponectin reduction in adipose tissue of ob/ob and dietary obese mice. Am J Physiology-Endocrinology Metab (2007) 293(4):E1118–28. doi:10.1152/ajpendo.00435.2007
16. Chakhtoura, M, Haber, R, Ghezzawi, M, Rhayem, C, Tcheroyan, R, and Mantzoros, CS. Pharmacotherapy of obesity: an update on the available medications and drugs under investigation. eClinicalMedicine (2023) 58:101882. doi:10.1016/j.eclinm.2023.101882
17. Chao, AM, Quigley, KM, and Wadden, TA. Dietary interventions for obesity: clinical and mechanistic findings. J Clin Invest (2021) 131(1):e140065. doi:10.1172/jci140065
18. Lundgren, JR, Janus, C, Jensen, SBK, Juhl, CR, Olsen, LM, Christensen, RM, et al. Healthy weight loss maintenance with exercise, liraglutide, or both combined. N Engl J Med (2021) 384(18):1719–30. doi:10.1056/nejmoa2028198
19. Perdomo, CM, Cohen, RV, Sumithran, P, Clément, K, and Frühbeck, G. Contemporary medical, device, and surgical therapies for obesity in adults. The Lancet (2023) 401(10382):1116–30. doi:10.1016/s0140-6736(22)02403-5
20. Fabbiano, S, Suárez-Zamorano, N, Rigo, D, Veyrat-Durebex, C, Stevanovic Dokic, A, Colin, DJ, et al. Caloric restriction leads to browning of white adipose tissue through type 2 immune signaling. Cel Metab (2016) 24(3):434–46. doi:10.1016/j.cmet.2016.07.023
21. Golbidi, S, Daiber, A, Korac, B, Li, H, Essop, MF, and Laher, I. Health benefits of fasting and caloric restriction. Curr Diab Rep (2017) 17(12):123. doi:10.1007/s11892-017-0951-7
22. Yu, Q, Zou, L, Kong, Z, and Yang, L. Cognitive impact of calorie restriction: a narrative review. J Am Med Directors Assoc (2020) 21(10):1394–401. doi:10.1016/j.jamda.2020.05.047
23. Dorling, JL, Martin, CK, and Redman, LM. Calorie restriction for enhanced longevity: the role of novel dietary strategies in the present obesogenic environment. Ageing Res Rev (2020) 64:101038. doi:10.1016/j.arr.2020.101038
24. Dansinger, ML, Gleason, JA, Griffith, JL, Selker, HP, and Schaefer, EJ. Comparison of the atkins, ornish, weight watchers, and zone diets for weight loss and heart disease risk ReductionA randomized trial. JAMA (2005) 293(1):43–53. doi:10.1001/jama.293.1.43
25. Li, M, Wang, S, Li, Y, Zhao, M, Kuang, J, Liang, D, et al. Gut microbiota-bile acid crosstalk contributes to the rebound weight gain after calorie restriction in mice. Nat Commun (2022) 13(1):2060. doi:10.1038/s41467-022-29589-7
26. Kim, KH, Kim, YH, Son, JE, Lee, JH, Kim, S, Choe, MS, et al. Intermittent fasting promotes adipose thermogenesis and metabolic homeostasis via VEGF-mediated alternative activation of macrophage. Cell Res (2017) 27(11):1309–26. doi:10.1038/cr.2017.126
27. Liu, B, Page, AJ, Hatzinikolas, G, Chen, M, Wittert, GA, and Heilbronn, LK. Intermittent fasting improves glucose tolerance and promotes adipose tissue remodeling in male mice fed a high-fat diet. Endocrinology (2019) 160(1):169–80. doi:10.1210/en.2018-00701
28. Liu, B, Page, AJ, Hutchison, AT, Wittert, GA, and Heilbronn, LK. Intermittent fasting increases energy expenditure and promotes adipose tissue browning in mice. Nutrition (2019) 66:38–43. doi:10.1016/j.nut.2019.03.015
29. Mattson, MP, Longo, VD, and Harvie, M. Impact of intermittent fasting on health and disease processes. Ageing Res Rev (2017) 39:46–58. doi:10.1016/j.arr.2016.10.005
30. Patterson, RE, and Sears, DD. Metabolic effects of intermittent fasting. Annu Rev Nutr (2017) 37(1):371–93. doi:10.1146/annurev-nutr-071816-064634
31. Varady, KA, Cienfuegos, S, Ezpeleta, M, and Gabel, K. Cardiometabolic benefits of intermittent fasting. Annu Rev Nutr (2021) 41(1):333–61. doi:10.1146/annurev-nutr-052020-041327
32. Catenacci, VA, Pan, Z, Ostendorf, D, Brannon, S, Gozansky, WS, Mattson, MP, et al. A randomized pilot study comparing zero-calorie alternate-day fasting to daily caloric restriction in adults with obesity. Obesity (2016) 24(9):1874–83. doi:10.1002/oby.21581
33. Duregon, E, Pomatto-Watson, LCDD, Bernier, M, Price, NL, and de Cabo, R. Intermittent fasting: from calories to time restriction. GeroScience (2021) 43(3):1083–92. doi:10.1007/s11357-021-00335-z
34. Ostendorf, DM, Caldwell, AE, Zaman, A, Pan, Z, Bing, K, Wayland, LT, et al. Comparison of weight loss induced by daily caloric restriction versus intermittent fasting (DRIFT) in individuals with obesity: study protocol for a 52-week randomized clinical trial. Trials (2022) 23:718. doi:10.1186/s13063-022-06523-2
35. Parvaresh, A, Razavi, R, Abbasi, B, Yaghoobloo, K, Hassanzadeh, A, Mohammadifard, N, et al. Modified alternate-day fasting vs. calorie restriction in the treatment of patients with metabolic syndrome: a randomized clinical trial. Complement Therapies Med (2019) 47:102187. doi:10.1016/j.ctim.2019.08.021
36. Longo, VD, Di Tano, M, Mattson, MP, and Guidi, N. Intermittent and periodic fasting, longevity and disease. Nat Aging (2021) 1(1):47–59. doi:10.1038/s43587-020-00013-3
37. Scholtens, EL, Krebs, JD, Corley, BT, and Hall, RM. Intermittent fasting 5:2 diet: what is the macronutrient and micronutrient intake and composition? Clin Nutr (2020) 39(11):3354–60. doi:10.1016/j.clnu.2020.02.022
38. Chaix, A, Zarrinpar, A, Miu, P, and Panda, S. Time-restricted feeding is a preventative and therapeutic intervention against diverse nutritional challenges. Cel Metab (2014) 20(6):991–1005. doi:10.1016/j.cmet.2014.11.001
39. Sutton, EF, Beyl, R, Early, KS, Cefalu, WT, Ravussin, E, and Peterson, CM. Early time-restricted feeding improves insulin sensitivity, blood pressure, and oxidative stress even without weight loss in men with prediabetes. Cel Metab (2018) 27(6):1212–21.e3. doi:10.1016/j.cmet.2018.04.010
40. Xie, Z, Sun, Y, Ye, Y, Hu, D, Zhang, H, He, Z, et al. Randomized controlled trial for time-restricted eating in healthy volunteers without obesity. Nat Commun (2022) 13(1):1003. doi:10.1038/s41467-022-28662-5
41. Lessan, N, and Ali, T. Energy metabolism and intermittent fasting: the ramadan perspective. Nutrients (2019) 11(5):1192. doi:10.3390/nu11051192
42. Fernando, HA, Zibellini, J, Harris, RA, Seimon, RV, and Sainsbury, A. Effect of ramadan fasting on weight and body composition in healthy non-athlete adults: a systematic review and meta-analysis. Nutrients (2019) 11(2):478. doi:10.3390/nu11020478
43. Kul, S, Savaş, E, Öztürk, ZA, and Karadağ, G. Does ramadan fasting alter body weight and blood lipids and fasting blood glucose in a healthy population? A meta-analysis. J Relig Health (2014) 53(3):929–42. doi:10.1007/s10943-013-9687-0
44. Dedual, MA, Wueest, S, Borsigova, M, and Konrad, D. Intermittent fasting improves metabolic flexibility in short-term high-fat diet-fed mice. Am J Physiology-Endocrinology Metab (2019) 317(5):E773–82. doi:10.1152/ajpendo.00187.2019
45. Halberg, N, Henriksen, M, Söderhamn, N, Stallknecht, B, Ploug, T, Schjerling, P, et al. Effect of intermittent fasting and refeeding on insulin action in healthy men. J Appl Physiol (2005) 99(6):2128–36. doi:10.1152/japplphysiol.00683.2005
46. Hirschey, MD, Shimazu, T, Goetzman, E, Jing, E, Schwer, B, Lombard, DB, et al. SIRT3 regulates mitochondrial fatty-acid oxidation by reversible enzyme deacetylation. Nature (2010) 464(7285):121–5. doi:10.1038/nature08778
47. Hu, X, Xia, K, Dai, M, Han, X, Yuan, P, Liu, J, et al. Intermittent fasting modulates the intestinal microbiota and improves obesity and host energy metabolism. Npj Biofilms Microbiomes (2023) 9(1):19–9. doi:10.1038/s41522-023-00386-4
48. Martinez-Lopez, N, Tarabra, E, Toledo, M, Garcia-Macia, M, Sahu, S, Coletto, L, et al. System-wide benefits of intermeal fasting by autophagy. Cel Metab (2017) 26(6):856–71.e5. doi:10.1016/j.cmet.2017.09.020
49. Li, G, Xie, C, Lu, S, Nichols, RG, Tian, Y, Li, L, et al. Intermittent fasting promotes white adipose browning and decreases obesity by shaping the gut microbiota. Cel Metab (2017) 26(4):801–685.e4. doi:10.1016/j.cmet.2017.10.007
50. Wu, J, Boström, P, Sparks, LM, Ye, L, Choi, JH, Giang, AH, et al. Beige adipocytes are a distinct type of thermogenic fat cell in mouse and human. Cell (2012) 150(2):366–76. doi:10.1016/j.cell.2012.05.016
51. Xu, G, Ji, C, Song, G, Zhao, C, Shi, C, Song, L, et al. MiR-26b modulates insulin sensitivity in adipocytes by interrupting the PTEN/PI3K/AKT pathway. Int J Obes (2015) 39(10):1523–30. doi:10.1038/ijo.2015.95
52. Zhu, S, Sun, F, Li, W, Cao, Y, Wang, C, Wang, Y, et al. Apelin stimulates glucose uptake through the PI3K/Akt pathway and improves insulin resistance in 3T3-L1 adipocytes. Mol Cel Biochem (2011) 353(1):305–13. doi:10.1007/s11010-011-0799-0
53. Li, X, Wang, F, Xu, M, Howles, P, and Tso, P. ApoA-IV improves insulin sensitivity and glucose uptake in mouse adipocytes via PI3K-Akt Signaling. Sci Rep (2017) 7(1):41289. doi:10.1038/srep41289
54. Xia, W, Pessentheiner, AR, Hofer, DC, Amor, M, Schreiber, R, Schoiswohl, G, et al. Loss of ABHD15 impairs the anti-lipolytic action of insulin by altering PDE3B stability and contributes to insulin resistance. Cel Rep (2018) 23(7):1948–61. doi:10.1016/j.celrep.2018.04.055
55. Hatting, M, Rines, AK, Luo, C, Tabata, M, Sharabi, K, Hall, JA, et al. Adipose tissue CLK2 promotes energy expenditure during high fat diet intermittent fasting. Cel Metab (2017) 25(2):428–37. doi:10.1016/j.cmet.2016.12.007
56. Li, T, Chen, K, Liu, G, Huang, LP, Chen, L, Wang, QW, et al. Calorie restriction prevents the development of insulin resistance and impaired lipid metabolism in gestational diabetes offspring. Pediatr Res (2017) 81(4):663–71. doi:10.1038/pr.2016.273
57. Yan, J, Wang, C, Jin, Y, Meng, Q, Liu, Q, Liu, Z, et al. Catalpol ameliorates hepatic insulin resistance in type 2 diabetes through acting on AMPK/NOX4/PI3K/AKT pathway. Pharmacol Res (2018) 130:466–80. doi:10.1016/j.phrs.2017.12.026
58. Zhang, Y, Gao, F, Gong, H, Fu, Y, Liu, B, Qin, X, et al. Intermittent fasting attenuates obesity-related atrial fibrillation via SIRT3-mediated insulin resistance mitigation. Biochim Biophys Acta (Bba) - Mol Basis Dis (2023) 1869(4):166638. doi:10.1016/j.bbadis.2023.166638
59. Katare, RG, Kakinuma, Y, Arikawa, M, Yamasaki, F, and Sato, T. Chronic intermittent fasting improves the survival following large myocardial ischemia by activation of BDNF/VEGF/PI3K signaling pathway. J Mol Cell Cardiol (2009) 46(3):405–12. doi:10.1016/j.yjmcc.2008.10.027
60. Song, NJ, Chang, SH, Kim, S, Panic, V, Jang, BH, Yun, UJ, et al. PI3Ka-Akt1-mediated Prdm4 induction in adipose tissue increases energy expenditure, inhibits weight gain, and improves insulin resistance in diet-induced obese mice. Cell Death Dis (2018) 9(9):876–13. doi:10.1038/s41419-018-0904-3
61. Castello, L, Maina, M, Testa, G, Cavallini, G, Biasi, F, Donati, A, et al. Alternate-day fasting reverses the age-associated hypertrophy phenotype in rat heart by influencing the ERK and PI3K signaling pathways. Mech Ageing Dev (2011) 132(6):305–14. doi:10.1016/j.mad.2011.06.006
62. Li, W, Wu, M, Zhang, Y, Wei, X, Zang, J, Liu, Y, et al. Intermittent fasting promotes adult hippocampal neuronal differentiation by activating GSK-3β in 3xTg-AD mice. J Neurochem (2020) 155(6):697–713. doi:10.1111/jnc.15105
63. Dakic, T, Jevdjovic, T, Djordjevic, J, and Vujovic, P. Short-term fasting differentially regulates PI3K/AkT/mTOR and ERK signalling in the rat hypothalamus. Mech Ageing Dev (2020) 192:111358. doi:10.1016/j.mad.2020.111358
64. Houtkooper, RH, Pirinen, E, and Auwerx, J. Sirtuins as regulators of metabolism and healthspan. Nat Rev Mol Cel Biol (2012) 13(4):225–38. doi:10.1038/nrm3293
65. Wu, QJ, Zhang, TN, Chen, HH, Yu, XF, Lv, JL, Liu, YY, et al. The sirtuin family in health and disease. Signal Transduction Targeted Ther (2022) 7(1):402–74. doi:10.1038/s41392-022-01257-8
66. Kuang, J, Zhang, Y, Liu, Q, Shen, J, Pu, S, Cheng, S, et al. Fat-specific Sirt6 ablation sensitizes mice to high-fat diet–induced obesity and insulin resistance by inhibiting lipolysis. Diabetes (2017) 66(5):1159–71. doi:10.2337/db16-1225
67. Wu, D, Bang, IH, Park, BH, and Bae, EJ. Loss of Sirt6 in adipocytes impairs the ability of adipose tissue to adapt to intermittent fasting. Exp Mol Med (2021) 53(9):1298–306. doi:10.1038/s12276-021-00664-1
68. Cohen, HY, Miller, C, Bitterman, KJ, Wall, NR, Hekking, B, Kessler, B, et al. Calorie restriction promotes mammalian cell survival by inducing the SIRT1 deacetylase. Science (2004) 305(5682):390–2. doi:10.1126/science.1099196
69. Pedersen, SB, Ølholm, J, Paulsen, SK, Bennetzen, MF, and Richelsen, B. Low Sirt1 expression, which is upregulated by fasting, in human adipose tissue from obese women. Int J Obes (2008) 32(8):1250–5. doi:10.1038/ijo.2008.78
70. Qiang, L, Wang, L, Kon, N, Zhao, W, Lee, S, Zhang, Y, et al. Brown remodeling of white adipose tissue by SirT1-dependent deacetylation of pparγ. Cell (2012) 150(3):620–32. doi:10.1016/j.cell.2012.06.027
71. Boutant, M, Kulkarni, SS, Joffraud, M, Raymond, F, Métairon, S, Descombes, P, et al. SIRT1 gain of function does not mimic or enhance the adaptations to intermittent fasting. Cel Rep (2016) 14(9):2068–75. doi:10.1016/j.celrep.2016.02.007
72. Yamagata, K, Mizumoto, T, and Yoshizawa, T. The emerging role of SIRT7 in glucose and lipid metabolism. Cells (2023) 13(1):48. doi:10.3390/cells13010048
73. Yoshizawa, T, Sato, Y, Sobuz, SU, Mizumoto, T, Tsuyama, T, Karim, MF, et al. SIRT7 suppresses energy expenditure and thermogenesis by regulating brown adipose tissue functions in mice. Nat Commun (2022) 13:7439. doi:10.1038/s41467-022-35219-z
74. Tang, X, Li, G, Shi, L, Su, F, Qian, M, Liu, Z, et al. Combined intermittent fasting and ERK inhibition enhance the anti-tumor effects of chemotherapy via the GSK3β-SIRT7 axis. Nat Commun (2021) 12:5058. doi:10.1038/s41467-021-25274-3
75. Saxton, RA, and Sabatini, DM. mTOR signaling in growth, metabolism, and disease. Cell (2017) 168(6):960–76. doi:10.1016/j.cell.2017.02.004
76. Bakan, I, and Laplante, M. Connecting mTORC1 signaling to SREBP-1 activation. Curr Opin Lipidol (2012) 23(3):226–34. doi:10.1097/mol.0b013e328352dd03
77. Chakrabarti, P, English, T, Shi, J, Smas, CM, and Kandror, KV. Mammalian target of rapamycin complex 1 suppresses lipolysis, stimulates lipogenesis, and promotes fat storage. Diabetes (2010) 59(4):775–81. doi:10.2337/db09-1602
78. Cai, H, Dong, LQ, and Liu, F. Recent advances in adipose mTOR signaling and function: therapeutic prospects. Trends Pharmacol Sci (2016) 37(4):303–17. doi:10.1016/j.tips.2015.11.011
79. Polak, P, Cybulski, N, Feige, JN, Auwerx, J, Rüegg, MA, and Hall, MN. Adipose-specific knockout of raptor results in lean mice with enhanced mitochondrial respiration. Cel Metab (2008) 8(5):399–410. doi:10.1016/j.cmet.2008.09.003
80. Godar, RJ, Ma, X, Liu, H, Murphy, JT, Weinheimer, CJ, Kovacs, A, et al. Repetitive stimulation of autophagy-lysosome machinery by intermittent fasting preconditions the myocardium to ischemia-reperfusion injury. Autophagy (2015) 11(9):1537–60. doi:10.1080/15548627.2015.1063768
81. Ma, X, Mani, K, Liu, H, Kovacs, A, Murphy, JT, Foroughi, L, et al. Transcription factor EB activation rescues advanced αb-crystallin mutation-induced cardiomyopathy by normalizing desmin localization. J Am Heart Assoc (2019) 8(4):e010866. doi:10.1161/jaha.118.010866
82. Martina, JA, Chen, Y, Gucek, M, and Puertollano, R. MTORC1 functions as a transcriptional regulator of autophagy by preventing nuclear transport of TFEB. Autophagy (2012) 8(6):903–14. doi:10.4161/auto.19653
83. Mukai, R, Zablocki, D, and Sadoshima, J. Intermittent fasting reverses an advanced form of cardiomyopathy. J Am Heart Assoc (2019) 8(4):e011863. doi:10.1161/jaha.118.011863
84. Sun, Y, Ni, X, Cheng, S, Yu, X, Jin, X, Chen, L, et al. Acteoside improves adipocyte browning by CDK6-mediated mTORC1-TFEB pathway. Biochim Biophys Acta (Bba) - Mol Cel Biol Lipids (2023) 1868(9):159364. doi:10.1016/j.bbalip.2023.159364
85. Hardie, DG, Schaffer, BE, and Brunet, A. AMPK: an energy-sensing pathway with multiple inputs and outputs. Trends Cel Biol (2016) 26(3):190–201. doi:10.1016/j.tcb.2015.10.013
86. Kajita, K, Mune, T, Ikeda, T, Matsumoto, M, Uno, Y, Sugiyama, C, et al. Effect of fasting on PPARγ and AMPK activity in adipocytes. Diabetes Res Clin Pract (2008) 81(2):144–9. doi:10.1016/j.diabres.2008.05.003
87. Liu, HW, and Chang, SJ. Moderate exercise suppresses NF-κB signaling and activates the SIRT1-AMPK-pgc1α Axis to attenuate muscle loss in diabetic db/db mice. Front Physiol (2018) 9:636. doi:10.3389/fphys.2018.00636
88. Wu, L, Zhang, L, Li, B, Jiang, H, Duan, Y, Xie, Z, et al. AMP-activated protein kinase (AMPK) regulates energy metabolism through modulating thermogenesis in adipose tissue. Front Physiol (2018) 9:122. doi:10.3389/fphys.2018.00122
89. Iwabu, M, Yamauchi, T, Okada-Iwabu, M, Sato, K, Nakagawa, T, Funata, M, et al. Adiponectin and AdipoR1 regulate PGC-1α and mitochondria by Ca2+ and AMPK/SIRT1. Nature (2010) 464(7293):1313–9. doi:10.1038/nature08991
90. Mottillo, EP, Desjardins, EM, Crane, JD, Smith, BK, Green, AE, Ducommun, S, et al. Lack of adipocyte AMPK exacerbates insulin resistance and hepatic steatosis through Brown and beige adipose tissue function. Cel Metab (2016) 24(1):118–29. doi:10.1016/j.cmet.2016.06.006
91. Tao, R, Gong, J, Luo, X, Zang, M, Guo, W, Wen, R, et al. AMPK exerts dual regulatory effects on the PI3K pathway. J Mol Signaling (2010) 5(1):1. doi:10.1186/1750-2187-5-1
92. Ruderman, NB, Carling, D, Prentki, M, and Cacicedo, JM. AMPK, insulin resistance, and the metabolic syndrome. J Clin Invest (2013) 123(7):2764–72. doi:10.1172/jci67227
93. Czech, MP. Insulin action and resistance in obesity and type 2 diabetes. Nat Med (2017) 23(7):804–14. doi:10.1038/nm.4350
94. Caron, A, Richard, D, and Laplante, M. The roles of mTOR complexes in lipid metabolism. Annu Rev Nutr (2015) 35(1):321–48. doi:10.1146/annurev-nutr-071714-034355
95. Kjøbsted, R, Roll, JLW, Jørgensen, NO, Birk, JB, Foretz, M, Viollet, B, et al. AMPK and TBC1D1 regulate muscle glucose uptake after, but not during, exercise and contraction. Diabetes (2019) 68(7):1427–40. doi:10.2337/db19-0050
96. Kliewer, KL, Ke, JY, Tian, M, Cole, RM, Andridge, RR, and Belury, MA. Adipose tissue lipolysis and energy metabolism in early cancer cachexia in mice. Cancer Biol Ther (2015) 16(6):886–97. doi:10.4161/15384047.2014.987075
97. Kazmirczak, F, Hartweck, LM, Vogel, NT, Mendelson, JB, Park, AK, Raveendran, RM, et al. Intermittent fasting activates AMP-kinase to restructure right ventricular lipid metabolism and microtubules. JACC: Basic Translational Sci (2023) 8(3):239–54. doi:10.1016/j.jacbts.2022.12.001
98. Park, M, Lyons, J, Oh, H, Yu, Y, Woltering, E, Greenway, F, et al. Enterostatin inhibition of angiogenesis: possible role of pAMPK and vascular endothelial growth factor A (VEGF-A). Int J Obes (2008) 32(6):922–9. doi:10.1038/ijo.2008.16
99. Nawaz, A, Mehmood, A, Kanatani, Y, Kado, T, Igarashi, Y, Takikawa, A, et al. Sirt1 activator induces proangiogenic genes in preadipocytes to rescue insulin resistance in diet-induced obese mice. Sci Rep (2018) 8(1):11370. doi:10.1038/s41598-018-29773-0
100. Jäger, S, Handschin, C, St.-Pierre, J, and Spiegelman, BM. AMP-activated protein kinase (AMPK) action in skeletal muscle via direct phosphorylation of PGC-1α. Proc Natl Acad Sci U S A (2007) 104(29):12017–22. doi:10.1073/pnas.0705070104
101. Yan, M, Audet-Walsh, É, Manteghi, S, Dufour, CR, Walker, B, Baba, M, et al. Chronic AMPK activation via loss of FLCN induces functional beige adipose tissue through PGC-1α/ERRα. Genes Dev (2016) 30(9):1034–46. doi:10.1101/gad.281410.116
102. Arany, Z, Foo, SY, Ma, Y, Ruas, JL, Bommi-Reddy, A, Girnun, G, et al. HIF-independent regulation of VEGF and angiogenesis by the transcriptional coactivator PGC-1α. Nature (2008) 451(7181):1008–12. doi:10.1038/nature06613
103. Yang, X, Liu, Q, Li, Y, Tang, Q, Wu, T, Chen, L, et al. The diabetes medication canagliflozin promotes mitochondrial remodeling of adipocyte via the AMPK-Sirt1-Pgc-1α signalling pathway. Adipocyte (2020) 9(1):484–94. doi:10.1080/21623945.2020.1807850
104. Guo, Z, Wang, M, Ying, X, Yuan, J, Wang, C, Zhang, W, et al. Caloric restriction increases the resistance of aged heart to myocardial ischemia/reperfusion injury via modulating AMPK–SIRT1–PGC1a energy metabolism pathway. Sci Rep (2023) 13(1):2045. doi:10.1038/s41598-023-27611-6
105. Hua, L, Li, J, Feng, B, Jiang, D, Jiang, X, Luo, T, et al. Dietary intake regulates white adipose tissues angiogenesis via liver fibroblast growth factor 21 in male mice. Endocrinology (2021) 162(3):bqaa244. doi:10.1210/endocr/bqaa244
106. Abu-Odeh, M, Zhang, Y, Reilly, SM, Ebadat, N, Keinan, O, Valentine, JM, et al. FGF21 promotes thermogenic gene expression as an autocrine factor in adipocytes. Cel Rep (2021) 35(13):109331. doi:10.1016/j.celrep.2021.109331
107. Chau, MDL, Gao, J, Yang, Q, Wu, Z, and Gromada, J. Fibroblast growth factor 21 regulates energy metabolism by activating the AMPK–SIRT1–PGC-1α pathway. Proc Natl Acad Sci U S A (2010) 107(28):12553–8. doi:10.1073/pnas.1006962107
108. Seki, T, Hosaka, K, Lim, S, Fischer, C, Honek, J, Yang, Y, et al. Endothelial PDGF-CC regulates angiogenesis-dependent thermogenesis in beige fat. Nat Commun (2016) 7(1):12152. doi:10.1038/ncomms12152
109. Seki, T, Hosaka, K, Fischer, C, Lim, S, Andersson, P, Abe, M, et al. Ablation of endothelial VEGFR1 improves metabolic dysfunction by inducing adipose tissue browning. J Exp Med (2018) 215(2):611–26. doi:10.1084/jem.20171012
110. Corvera, S, Solivan-Rivera, J, and Yang Loureiro, Z. Angiogenesis in adipose tissue and obesity. Angiogenesis (2022) 25(4):439–53. doi:10.1007/s10456-022-09848-3
111. Liu, G, Bi, Y, Shen, B, Yang, H, Zhang, Y, Wang, X, et al. SIRT1 limits the function and fate of myeloid-derived suppressor cells in tumors by orchestrating HIF-1α-dependent glycolysis. Cancer Res (2014) 74(3):727–37. doi:10.1158/0008-5472.can-13-2584
112. Park, J, Kim, M, Sun, K, An, YA, Gu, X, and Scherer, PE. VEGF-A–Expressing adipose tissue shows rapid beiging and enhanced survival after transplantation and confers IL-4–independent metabolic improvements. Diabetes (2017) 66(6):1479–90. doi:10.2337/db16-1081
113. Ding, X, Luo, Y, Zhang, X, Zheng, H, Yang, X, Yang, X, et al. IL-33-driven ILC2/eosinophil Axis in fat is induced by sympathetic tone and suppressed by obesity. J Endocrinol (2016) 231(1):35–48. doi:10.1530/joe-16-0229
114. Lee, MW, Odegaard, JI, Mukundan, L, Qiu, Y, Molofsky, AB, Nussbaum, JC, et al. Activated type 2 innate lymphoid cells regulate beige fat biogenesis. Cell (2015) 160(1–2):74–87. doi:10.1016/j.cell.2014.12.011
115. Molofsky, AB, Nussbaum, JC, Liang, HE, Van Dyken, SJ, Cheng, LE, Mohapatra, A, et al. Innate lymphoid type 2 cells sustain visceral adipose tissue eosinophils and alternatively activated macrophages. J Exp Med (2013) 210(3):535–49. doi:10.1084/jem.20121964
116. Bartness, TJ, Shrestha, YB, Vaughan, CH, Schwartz, GJ, and Song, CK. Sensory and sympathetic nervous system control of white adipose tissue lipolysis. Mol Cell Endocrinol (2010) 318(1):34–43. doi:10.1016/j.mce.2009.08.031
117. Bartness, TJ, Liu, Y, Shrestha, YB, and Ryu, V. Neural innervation of white adipose tissue and the control of lipolysis. Front Neuroendocrinology (2014) 35(4):473–93. doi:10.1016/j.yfrne.2014.04.001
118. Migliorini, RH, Garofalo, MA, and Kettelhut, IC. Increased sympathetic activity in rat white adipose tissue during prolonged fasting. Am J Physiology-Regulatory, Integr Comp Physiol (1997) 272(2):R656–61. doi:10.1152/ajpregu.1997.272.2.r656
119. Giordano, A, Frontini, A, Murano, I, Tonello, C, Marino, MA, Carruba, MO, et al. Regional-dependent increase of sympathetic innervation in rat white adipose tissue during prolonged fasting. J Histochem Cytochem (2005) 53(6):679–87. doi:10.1369/jhc.4a6566.2005
120. Zanquetta, MM, Nascimento, MEC, Mori, RCT, D’Agord Schaan, B, Young, ME, and Machado, UF. Participation of β-adrenergic activity in modulation of GLUT4 expression during fasting and refeeding in rats. Metabolism (2006) 55(11):1538–45. doi:10.1016/j.metabol.2006.06.026
121. Klein, S, Peters, EJ, Holland, OB, and Wolfe, RR. Effect of short- and long-term beta-adrenergic blockade on lipolysis during fasting in humans. Am J Physiology-Endocrinology Metab (1989) 257(1):E65–73. doi:10.1152/ajpendo.1989.257.1.e65
122. Harney, DJ, Cielesh, M, Chu, R, Cooke, KC, James, DE, Stöckli, J, et al. Proteomics analysis of adipose depots after intermittent fasting reveals visceral fat preservation mechanisms. Cel Rep (2021) 34(9):108804. doi:10.1016/j.celrep.2021.108804
123. Zhao, Y, Li, X, Yang, L, Eckel-Mahan, K, Tong, Q, Gu, X, et al. Transient overexpression of vascular endothelial growth factor A in adipose tissue promotes energy expenditure via activation of the sympathetic nervous system. Mol Cell Biol (2018) 38(22):e00242–18. doi:10.1128/mcb.00242-18
124. Speliotes, EK, Willer, CJ, Berndt, SI, Monda, KL, Thorleifsson, G, Jackson, AU, et al. Association analyses of 249,796 individuals reveal 18 new loci associated with body mass index. Nat Genet (2010) 42(11):937–48. doi:10.1038/ng.686
125. Thorleifsson, G, Walters, GB, Gudbjartsson, DF, Steinthorsdottir, V, Sulem, P, Helgadottir, A, et al. Genome-wide association yields new sequence variants at seven loci that associate with measures of obesity. Nat Genet (2009) 41(1):18–24. doi:10.1038/ng.274
126. Nigro, P, Vamvini, M, Yang, J, Caputo, T, Ho, LL, Carbone, NP, et al. Exercise training remodels inguinal white adipose tissue through adaptations in innervation, vascularization, and the extracellular matrix. Cel Rep (2023) 42(4):112392. doi:10.1016/j.celrep.2023.112392
127. Hayes, NVL, Newsam, RJ, Baines, AJ, and Gullick, WJ. Characterization of the cell membrane-associated products of the Neuregulin 4 gene. Oncogene (2008) 27(5):715–20. doi:10.1038/sj.onc.1210689
128. Zeng, F, Wang, Y, Kloepfer, LA, Wang, S, and Harris, RC. ErbB4 deletion predisposes to development of metabolic syndrome in mice. Am J Physiology-Endocrinology Metab (2018) 315(4):E583–93. doi:10.1152/ajpendo.00166.2018
129. Meng, X, Qian, X, Ding, X, Wang, W, Yin, X, Zhuang, G, et al. Eosinophils regulate intra-adipose axonal plasticity. Proc Natl Acad Sci USA (2022) 119(3):e2112281119. doi:10.1073/pnas.2112281119
130. Cao, Y, Wang, H, and Zeng, W. Whole-tissue 3D imaging reveals intra-adipose sympathetic plasticity regulated by NGF-TrkA signal in cold-induced beiging. Protein Cell (2018) 9(6):527–39. doi:10.1007/s13238-018-0528-5
131. Wang, YN, Tang, Y, He, Z, Ma, H, Wang, L, Liu, Y, et al. Slit3 secreted from M2-like macrophages increases sympathetic activity and thermogenesis in adipose tissue. Nat Metab (2021) 3(11):1536–51. doi:10.1038/s42255-021-00482-9
132. Ealey, KN, Togo, J, Lee, JH, Patel, Y, Kim, JR, Park, SY, et al. Intermittent fasting promotes rejuvenation of immunosenescent phenotypes in aged adipose tissue. GeroScience (2024) 46(3):3457–70. doi:10.1007/s11357-024-01093-4
133. Brestoff, JR, Kim, BS, Saenz, SA, Stine, RR, Monticelli, LA, Sonnenberg, GF, et al. Group 2 innate lymphoid cells promote beiging of white adipose tissue and limit obesity. Nature (2015) 519(7542):242–6. doi:10.1038/nature14115
134. Lumeng, CN, DelProposto, JB, Westcott, DJ, and Saltiel, AR. Phenotypic switching of adipose tissue macrophages with obesity is generated by spatiotemporal differences in macrophage subtypes. Diabetes (2008) 57(12):3239–46. doi:10.2337/db08-0872
135. Molofsky, AB, Van Gool, F, Liang, HE, Van Dyken, SJ, Nussbaum, JC, Lee, J, et al. Interleukin-33 and interferon-γ counter-regulate group 2 innate lymphoid cell activation during immune perturbation. Immunity (2015) 43(1):161–74. doi:10.1016/j.immuni.2015.05.019
136. Duregotti, E, Reumiller, CM, Mayr, U, Hasman, M, Schmidt, LE, Burnap, SA, et al. Reduced secretion of neuronal growth regulator 1 contributes to impaired adipose-neuronal crosstalk in obesity. Nat Commun (2022) 13(1):7269. doi:10.1038/s41467-022-34846-w
137. Kim, SJ, Tang, T, Abbott, M, Viscarra, JA, Wang, Y, and Sul, HS. AMPK phosphorylates desnutrin/ATGL and hormone-sensitive lipase to regulate lipolysis and fatty acid oxidation within adipose tissue. Mol Cell Biol (2016) 36(14):1961–76. doi:10.1128/mcb.00244-16
138. Gauthier, MS, Miyoshi, H, Souza, SC, Cacicedo, JM, Saha, AK, Greenberg, AS, et al. AMP-Activated protein kinase is activated as a consequence of lipolysis in the adipocyte. J Biol Chem (2008) 283(24):16514–24. doi:10.1074/jbc.m708177200
139. Borgundvaag, E, Mak, J, and Kramer, CK. Metabolic impact of intermittent fasting in patients with type 2 diabetes mellitus: a systematic review and meta-analysis of interventional studies. J Clin Endocrinol Metab (2021) 106(3):902–11. doi:10.1210/clinem/dgaa926
140. Meng, H, Zhu, L, Kord-Varkaneh, H, O Santos, H, Tinsley, GM, and Fu, P. Effects of intermittent fasting and energy-restricted diets on lipid profile: a systematic review and meta-analysis. Nutrition (2020) 77:110801. doi:10.1016/j.nut.2020.110801
141. Patikorn, C, Roubal, K, Veettil, SK, Chandran, V, Pham, T, Lee, YY, et al. Intermittent fasting and obesity-related health outcomes: an umbrella review of meta-analyses of randomized clinical trials. JAMA Netw Open (2021) 4(12):e2139558. doi:10.1001/jamanetworkopen.2021.39558
142. Sun, ML, Yao, W, Wang, XY, Gao, S, Varady, KA, Forslund, SK, et al. Intermittent fasting and health outcomes: an umbrella review of systematic reviews and meta-analyses of randomised controlled trials. eClinicalMedicine (2024) 70:102519. doi:10.1016/j.eclinm.2024.102519
143. Wang, X, Yang, Q, Liao, Q, Li, M, Zhang, P, Santos, HO, et al. Effects of intermittent fasting diets on plasma concentrations of inflammatory biomarkers: a systematic review and meta-analysis of randomized controlled trials. Nutrition (2020) 79–80:110974. doi:10.1016/j.nut.2020.110974
144. Jebeile, H, Gow, ML, Lister, NB, Mosalman Haghighi, M, Ayer, J, Cowell, CT, et al. Intermittent energy restriction is a feasible, effective, and acceptable intervention to treat adolescents with obesity. J Nutr (2019) 149(7):1189–97. doi:10.1093/jn/nxz049
145. Deng, Y, Yang, X, Ye, X, Yuan, Y, Zhang, Y, Teng, F, et al. Alternate day fasting aggravates atherosclerosis through the suppression of hepatic ATF3 in Apoe−/− mice. Life Metab (2024) 3(3):loae009. doi:10.1093/lifemeta/loae009
146. Park, S, Yoo, KM, Hyun, JS, and Kang, S. Intermittent fasting reduces body fat but exacerbates hepatic insulin resistance in young rats regardless of high protein and fat diets. J Nutr Biochem (2017) 40:14–22. doi:10.1016/j.jnutbio.2016.10.003
147. Arriola Apelo, SI, Pumper, CP, Baar, EL, Cummings, NE, and Lamming, DW. Intermittent administration of rapamycin extends the life span of female C57bl/6J mice. Journals Gerontol Ser A: Biol Sci Med Sci (2016) 71(7):876–81. doi:10.1093/gerona/glw064
148. Alfaras, I, Mitchell, SJ, Mora, H, Lugo, DR, Warren, A, Navas-Enamorado, I, et al. Health benefits of late-onset metformin treatment every other week in mice. Npj Aging Mech Dis (2017) 3(1):16–3. doi:10.1038/s41514-017-0018-7
149. Masarwa, R, Brunetti, VC, Aloe, S, Henderson, M, Platt, RW, and Filion, KB. Efficacy and safety of metformin for obesity: a systematic review. Pediatrics (2021) 147(3):e20201610. doi:10.1542/peds.2020-1610
150. Yerevanian, A, and Soukas, AA. Metformin: mechanisms in human obesity and weight loss. Curr Obes Rep (2019) 8(2):156–64. doi:10.1007/s13679-019-00335-3
151. Carling, D. AMPK signalling in health and disease. Curr Opin Cel Biol (2017) 45:31–7. doi:10.1016/j.ceb.2017.01.005
152. Kim, J, Yang, G, Kim, Y, Kim, J, and Ha, J. AMPK activators: mechanisms of action and physiological activities. Exp Mol Med (2016) 48(4):e224. doi:10.1038/emm.2016.16
153. Soukas, AA, Hao, H, and Wu, L. Metformin as anti-aging therapy: is it for everyone? Trends Endocrinol Metab (2019) 30(10):745–55. doi:10.1016/j.tem.2019.07.015
154. Steinberg, GR, and Hardie, DG. New insights into activation and function of the AMPK. Nat Rev Mol Cel Biol (2023) 24(4):255–72. doi:10.1038/s41580-022-00547-x
155. McCreight, LJ, Bailey, CJ, and Pearson, ER. Metformin and the gastrointestinal tract. Diabetologia (2016) 59:426–35. doi:10.1007/s00125-015-3844-9
Glossary
Keywords: obesity, intermittent fasting, adipose tissue remodeling, angiogenesis, sympathetic innervation
Citation: Vo N, Zhang Q and Sung H-K (2024) From fasting to fat reshaping: exploring the molecular pathways of intermittent fasting-induced adipose tissue remodeling. J. Pharm. Pharm. Sci 27:13062. doi: 10.3389/jpps.2024.13062
Received: 30 March 2024; Accepted: 05 July 2024;
Published: 22 July 2024.
Edited by:
John Reyes Ussher, University of Alberta, CanadaCopyright © 2024 Vo, Zhang and Sung. This is an open-access article distributed under the terms of the Creative Commons Attribution License (CC BY). The use, distribution or reproduction in other forums is permitted, provided the original author(s) and the copyright owner(s) are credited and that the original publication in this journal is cited, in accordance with accepted academic practice. No use, distribution or reproduction is permitted which does not comply with these terms.
*Correspondence: Hoon-Ki Sung, aG9vbi1raS5zdW5nQHNpY2traWRzLmNh