- 1Faculty of Pharmacy and Pharmaceutical Sciences, University of Alberta, Edmonton, AB, Canada
- 2Alberta Diabetes Institute, University of Alberta, Edmonton, AB, Canada
- 3Cardiovascular Research Institute, University of Alberta, Edmonton, AB, Canada
Cardiovascular disease including diabetic cardiomyopathy (DbCM) represents the leading cause of death in people with diabetes. DbCM is defined as ventricular dysfunction in the absence of underlying vascular diseases and/or hypertension. The known molecular mediators of DbCM are multifactorial, including but not limited to insulin resistance, altered energy metabolism, lipotoxicity, endothelial dysfunction, oxidative stress, apoptosis, and autophagy. FoxO1, a prominent member of forkhead box O transcription factors, is involved in regulating various cellular processes in different tissues. Altered FoxO1 expression and activity have been associated with cardiovascular diseases in diabetic subjects. Herein we provide an overview of the role of FoxO1 in various molecular mediators related to DbCM, such as altered energy metabolism, lipotoxicity, oxidative stress, and cell death. Furthermore, we provide valuable insights into its therapeutic potential by targeting these perturbations to alleviate cardiomyopathy in settings of type 1 and type 2 diabetes.
Introduction
Diabetes has evolved exponentially, affects 463 million people worldwide, and prevalence is expected to increase to 700 million by 2045 [1]. Despite numerous advancements in the management of hyperglycemia, cardiovascular diseases including myocardial infarction or heart failure remain the number one cause of death in people with type 1 diabetes (T1D) or type 2 diabetes (T2D) [2]. Although macrovascular dysfunction, endothelial dysfunction, atherosclerosis, and hypertension are increased in diabetic individuals, the increased risk of heart failure is often independent of these comorbidities [3, 4]. Moreover, people with diabetes frequently develop an asymptomatic diastolic dysfunction, a hallmark of diabetic cardiomyopathy (DbCM) [5]. Although the definition of DbCM is still evolving, it is unequivocally considered as ventricular dysfunction with altered myocardial metabolism in the absence of underlying coronary artery diseases and/or hypertension in people with diabetes [5, 6]. Our understanding of pathological changes and molecular mediators of DbCM has greatly improved in last few decades [5], yet there is no approved therapy.
Forkhead box O (FoxO) transcription factors, including FoxO1, FoxO3, FoxO4, and FoxO6, have important roles in several signaling pathways involved in human health and diseases [7]. Out of these subtypes, FoxO1 and FoxO3 are known to be essential for the maintenance of cardiac health by having pivotal roles in the regulations of cellular processes [8]. Lately, with the availability of various pre-clinical models of DbCM, mounting evidence has shown that FoxO1 activity is upregulated in diabetic myocardium [9, 10]. It is also widely accepted that FoxO1 could contribute to the pathogenesis of DbCM via direct or indirect regulations of molecular targets involved in metabolism, oxidative stress, endothelial dysfunction, and apoptosis [10].
In this review, we will provide an overview of the pathology of DbCM and discuss the FoxO1-driven regulations of its key mediators. While our focus will primarily be DbCM in the context of T2D, we will also consider these aspects in the setting of T1D. Furthermore, we will interrogate whether FoxO1 could be a potential therapeutic target for the treatment of DbCM.
Diabetic cardiomyopathy
The DbCM was first described by Rubler and colleagues in 1972 through findings from an autopsy of four diabetic individuals with no sign of myocardial infarction but with left ventricular (LV) hypertrophy, gross cardiomegaly, and congestive heart failure [11]. These observations led to the very first definition of DbCM, a ventricular dysfunction in the absence of underlying coronary artery disease and/or hypertension in people with diabetes. Although the clinical phenotype of DbCM is still under active investigation, our understanding of it has greatly advanced by utilizing modern non-invasive imaging technology [12, 13]. The growing recognition of diastolic dysfunction and alterations in myocardial metabolism mainly an elevation in fatty acid oxidation and a reduction in glucose oxidation in early-stage T2D is reshaping perspectives of DbCM, re-terming or redefining it as “diabetic heart disease” [5] or “diastolic dysfunction with altered myocardial metabolism without other known causes of cardiomyopathy and/or hypertension” [6]. However, re-terming it as “diabetic heart disease” could mistakenly encompass all cardiovascular conditions linked to diabetes, not just those affecting the myocardium, but also vascular diseases. Moreover, diastolic dysfunction often lacks symptoms and remains undiagnosed in diabetic individuals until a noticeable decline occurs, yet its prevalence in T2D has been reported to range from 20 to 80% based on diagnostic criteria and patient group [14–17]. Indeed, we concur with these perspectives, particularly as diastolic dysfunction and DbCM are significant risk factors for the advancement of heart failure with preserved ejection fraction (HFpEF), which is quite prevalent in individuals with diabetes [18]. Although the advancements in the understanding of DbCM have been greatly appreciated in the last few decades [5, 19], it is still unclear why some individuals with diabetes develop HFpEF whereas others develop heart failure with reduced ejection fraction (HFrEF).
Our understanding of the various mechanisms that contribute to the pathology of DbCM has greatly enhanced with improved knowledge of animal models of obesity and insulin resistance (extensively reviewed by Heather et al. [20]). As of now, we are fully aware of several attenuated cellular processes identified within the myocardium of DbCM subjects. These include lipotoxicity, glucotoxicity, mitochondrial dysfunction, abnormal substrate metabolism, oxidative stress, inflammation, and abnormal calcium handling, many of which can lead to the death of cardiac cells, we encourage the reader to refer to the excellent reviews on this topic [5, 19, 21, 22]. Assessing how these factors individually affect diastolic dysfunction in T2D individuals is challenging due to their cross-talk. For instance, insulin resistance can alter metabolism, leading to mitochondrial dysfunction, oxidative stress, and lipotoxicity [23, 24]. Identifying the most effective target for improving diastolic dysfunction remains uncertain, highlighting the need for future studies to explore these mechanisms in T2D subjects.
Forkhead box O1 transcription factor (FoxO1)
The “Forkhead” protein was first identified in 1989 in Drosophila melanogaster as a transcriptional regulator containing a winged-helix DNA binding domain [25, 26]. Later in the 1990s, FoxO was identified as abnormal dauer formation-16 (DAF-16) in Caenorhabditis elegans and as forkhead in rhabdomyosarcoma (FKHR) in tumor tissues from eight patients with alveolar rhabdomyosarcomas [27, 28]. In humans, there are four FoxO proteins including FoxO1, FoxO3, FoxO4, and FoxO6 are known to be present in various tissues [29, 30]. Although FoxO6 was initially thought to be mainly in the brain, it is now known for a ubiquitous expression as well [31]. FoxO1/3/4/6 proteins through their conserved forkhead domain specifically recognize DAF-16 binding element (DBE) 5′-GTAAACAA-3′ and insulin-responsive element (IRE) 5´-(C/A)(A/C)AAA (C/T)AA-3′ to transcriptionally regulate the expression of genes (extensively reviewed in [32, 33]). FoxO’s nuclear transit and transcriptional activity are also regulated by various post-translational modifications such as phosphorylation, acetylation, O-glycosylation, methylation, and ubiquitination. Although several kinases (e.g., mitogen-activated protein kinases, c-Jun N-terminal kinases, cyclin-dependent kinase 2, nuclear factor κB, etc.) are known to be involved in the phosphorylation of FoxO1, protein kinase B (also known as AKT), a downstream target of insulin signaling have been considered a prime kinase which negatively regulates FoxO1 by phosphorylation and nuclear exclusion in the context of metabolism (Figure 1) [34, 35]. In the 21st century, a plethora of studies concluded the essential role of FoxO transcription factors in myocardial homeostasis through the regulation of cell proliferation, oxidative stress, energy metabolism, and cell death (extensively reviewed in [36, 37]). FoxO1, among other “O” subfamilies, has been considered the front-runner in controlling myocardial equilibrium in the settings of metabolic diseases (especially in DbCM) [10, 36, 38].
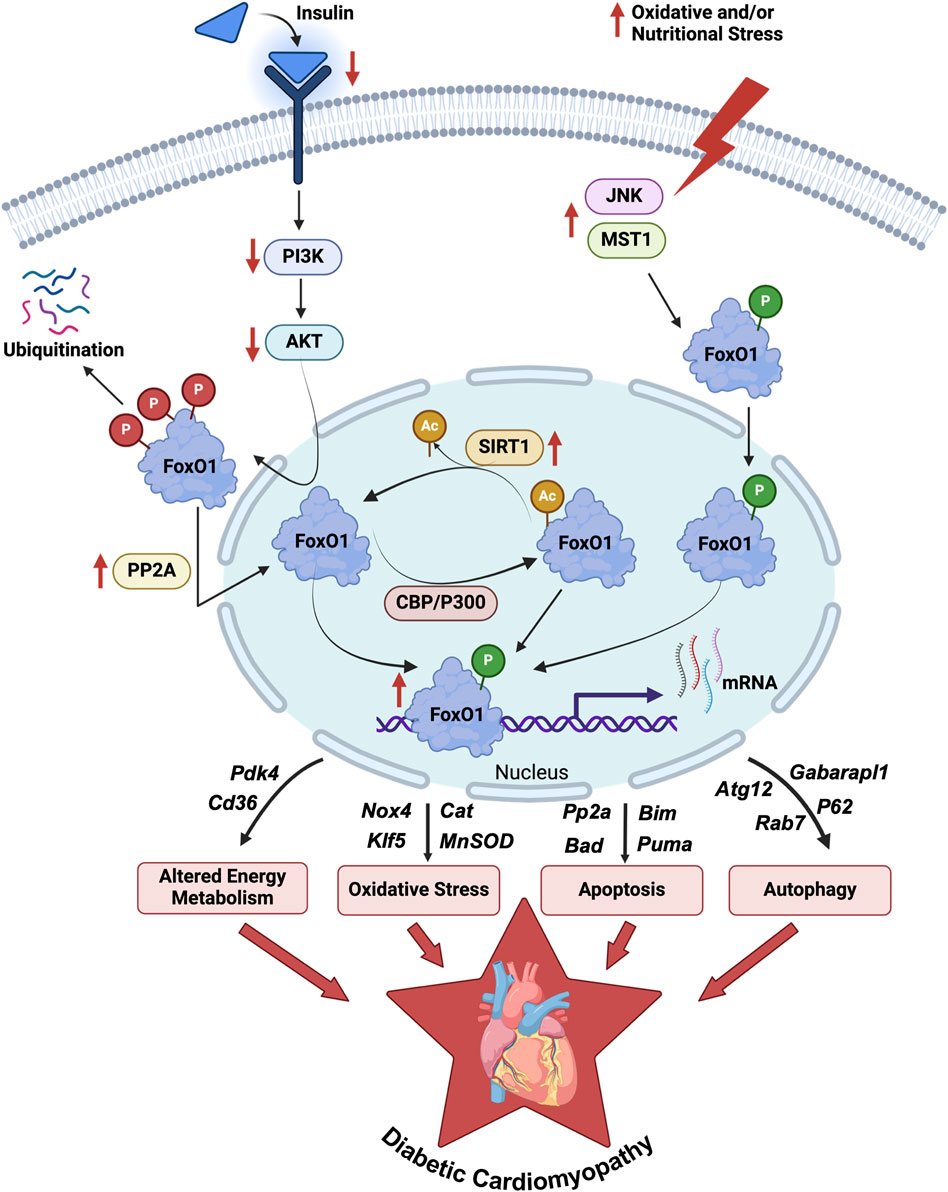
Figure 1. Regulations and role of FoxO1 in DbCM. An illustration depicts the regulation of FoxO1 activity via post-translational modifications such as phosphorylation, acetylation, and ubiquitination in the myocardium of diabetic individuals. The enhanced FoxO1 activity transcriptionally upregulates several genes (Table 1) involved in myocardial energy metabolism, oxidative stress regulations, apoptosis, and autophagy reported in preclinical and clinical studies of DbCM. DbCM, diabetic cardiomyopathy; FoxO1, Forkhead box O1 transcription factor; PI3K, Phosphoinositide 3-kinases; AKT, Protein kinase B; PP2A, Protein phosphatase 2A; CBP, cAMP-response element binding protein; P300, Histone acetyltransferase p300; SIRT1, Sirtuin-1; JNK, c-Jun N-terminal kinase; MST1, Macrophage stimulating 1; Pdk4, Pyruvate dehydrogenase kinase 4; Cd36, fatty acid translocase; Nox4, NADPH oxidase 4; Klf5, Kruppel-like factor 5; Cat, Catalase; MnSOD, Manganese superoxide dismutase; Bim, Bcl2 interacting mediator; Bad, Bcl2 antagonist of cell death; Puma, p53 upregulated modulator of apoptosis; Atg12, Autophagy related 12; Rab7, Rat sarcoma virus-related protein 7; Gabarapl1, Gamma-aminobutyric acid receptor-associated protein-like 1; P62, ubiquitin-binding protein p62; P, Phosphorylation (Red, AKT mediated; Green, JNK1/MST mediated); Ac, Acetylation.
FoxO1 in diabetic cardiomyopathy
The plethora of evidence suggests that the pathophysiology of DbCM [9, 39, 40], and ischemic heart disease [41] are linked to upregulated FoxO1 activity. Although there is no direct clinical evidence of FoxO1 activation during DbCM, its DNA binding sites are overrepresented in the promoter sequences of heart failure genes in isolated RNA from the myocardium of heart failure patients with either ischemic or idiopathic dilated cardiomyopathy [42]. Additionally, RNA sequencing data of human hearts with dilated cardiomyopathy showed an enriched FoxO1-binding motif, suggestive of enhanced transcriptional activity [43]. However, the contribution of cellular mechanisms associated with FoxO1 signaling in the pathogenesis of DbCM is not yet fully understood. The connection between FoxO1 activation and the pathogenesis of DbCM mainly stemmed from in vivo animal models and in vitro studies [9, 44]. In the case of insulin resistance and diabetes, reduced growth signals and increased stress signals lead to weaker nuclear exportation mechanisms for FoxO1, resulting in increased FoxO1 transcriptional activity in cardiomyocytes [36]. The increased transcriptional activity of FoxO1 precipitates shifts in gene expression, consequently inducing modifications in myocardial energy metabolism, lipotoxicity, oxidative stress, and cellular damage in diabetic myocardium (Figure 1; Table 1).
FoxO1 in metabolic abnormalities during diabetic cardiomyopathy
Numerous studies have consistently affirmed the idea that disruptions in myocardial glucose and fatty acid metabolism serve as primary triggers for cardiac dysfunction in diabetic conditions, a topic thoroughly reviewed by Heather et al. [6]. FoxO1 is involved in various pathways related to myocardial energy metabolism. Battiprolu et al. have shown that 25 weeks of high-fat diet (HFD) (60% kcal from lard) feeding to male C57BL/6J mice induces myocardial nuclear enrichment of FoxO1, leading to enhancement in myocardial triacylglycerol (TAG) content, LV hypertrophy, and cardiac systolic dysfunction, which was not apparent in HFD-fed cardiac-specific FoxO1 deficient mice [9]. In addition, enhanced FoxO1 nuclear compartmentalization contributed to elevations in myocardial pyruvate dehydrogenase (PDH) kinase 4 (Pdk4) transcription and impairment in PDH activity in the myocardial tissues of HFD-fed mice. Concurrently, we have shown that FoxO1 binds to the DBE sequence in the promoter of the Pdk4 gene to upregulate its expression in cardiomyocytes and reduce myocardial glucose oxidation rates [45]. As the glucose oxidation produces more ATP per mole of consumed oxygen than the fatty acid oxidation, reduced cardiac function correlated with higher oxygen consumption and lower cardiac efficiency in ob/ob mice with reduced glucose oxidation and increased fatty acid oxidation [56, 57]. Additionally, the increases in the myocardial delivery of fatty acids due to adaptive changes may lead to the uncoupling of the mitochondria, leading to a reduction in ATP production which aligns with the reduced cardiac performance. Moreover, recent studies from our lab targeting this FoxO1-Pdk4 axis using either AS1842856 (FoxO1 inhibitor) or cardiac-specific FoxO1 elimination alleviated diastolic dysfunction via increasing myocardial glucose oxidation rates in male mice subjected to experimental T2D via HFD supplementation for 10 weeks with a single dose (75 mg/kg) of streptozotocin (STZ) at 4th week [45, 46]. Similarly, male Sprague Dawley rats induced with T1D using STZ (65 mg/kg) and treated with AS1842856 demonstrated improved cardiac function using pressure-volume conductance catheters [47]. The isolated cardiomyocytes from these rats demonstrated increased oxygen consumption rates in the presence of glucose or pyruvate (indicative of increased glucose oxidation). These findings strongly advocate the role of FoxO1 in the reduction of glucose oxidation in the myocardium of diabetic mice with cardiac dysfunction.
In diabetic myocardium, decreases in glucose oxidation with elevated PDK4 expression often result in increases in fatty acid uptake and oxidation by following Randle’s cycle to meet constant energy demand, thereby promoting myocardial lipid accumulation [22]. Contrarily, mice with cardiac-specific overexpression of PDK4 were protected against HFD-induced myocardial lipid accumulation, likely due to adaptive metabolic re-programming for increased fatty acid oxidation [58]. However, Elevated myocardial TAG content-associated lipotoxicity has been verified in individuals with T2D and identified as an independent predictor of diastolic dysfunction [59]. A key early development in DbCM pathogenesis involves increased fatty acid transport across the sarcolemma, primarily controlled by fatty acid translocase (FAT/CD36) [60]. In conditions of lipid overload, the FoxO1/inducible NO-synthase (iNOS)/CD36 pathway was shown to mediate lipid accumulation in cardiomyocytes from adult male Wistar rats [48]. Palmitate exposure in isolated cardiomyocytes leads to a significant overload of intercellular TAG which triggers a chain reaction starting with the upregulation of FoxO1. The high expression of FoxO1 in the vascular endothelial cells leads to an overexpression of iNOS which activates the cell division control (Cdc) 42 protein through its nitration, resulting in cytoskeleton rearrangement. This process aids CD36 translocation and results in TAG accumulation in cardiomyocytes from adult male Wistar rats [48]. Moreover, Evogliptin (EVO), a dipeptidyl peptidase-4 (DPP-4) inhibitor known for its glucose-lowering effects in T2D, demonstrated the ability to prevent DbCM and associated lipotoxicity by suppressing CD36 protein expression and enhancing the phosphorylation of FoxO1 at Serine 256 position, indicative of its inactivation, in db/db mice [49]. Prostaglandin E receptor subtype 4 (EP4) is a G protein-coupled receptor (GPCR) highly expressed in cardiomyocytes. In a study involving mice supplemented with HFD for 8 weeks, EP4 was shown to protect against DbCM by modulating FoxO1/CD36-mediated fatty acid uptake [50]. The concentric hypertrophy and myocardial fibrosis in HFD-fed EP4-deficient mice converged with a reduction in myocardial fatty acid uptake and ATP production, which was corrected pharmacologically by activation of EP4. Thus, by targeting the FoxO1–CD36 axis, we could reduce the myocardial damage associated with lipotoxicity during diabetes.
FoxO1 in myocardial oxidative stress during diabetic cardiomyopathy
It is undebatable that hyperglycemia along with enhanced fatty acid oxidation and mitochondrial dysfunction contributes to oxidative stress by increasing reactive oxygen species (ROS) including superoxide and H2O2 levels in the diabetic myocardium [61]. FoxO1 has been known to play a dual role during oxidative stress regulation based on the cellular microenvironment and level of oxidative stress [37]. Recently, Krüppel-like factor (KLF) 5 directly transcriptionally regulated by FoxO1 was shown to cause oxidative stress via induction of NADPH oxidase (NOX) 4 expression, a major source of cytosolic ROS levels [62] in cardiomyocytes of STZ-induced T1D mice [51]. Cardiac-specific FoxO1 elimination remarkably reduced KLF5 expression and prevented oxidative stress and cardiac dysfunction, which was reverted by over-expression of FoxO1 or KLF5 in cardiomyocytes of T1D mice. Concurrently, Curcumin, a natural antioxidant, treatment in male Sprague-Dawley rats fed a high-glucose and HFD (40% fat, 41% carbohydrates, and 18% protein) and supplemented with STZ (60 mg/kg; 3 days) was shown to alleviate oxidative stress and DbCM by FoxO1 modulation via sirtuin 1 (Sirt1) and phosphoinositide 3-kinases (PI3K)-AKT signaling pathways [52]. Moreover, high glucose upregulated thioredoxin (Trx) interacting protein (Txnip) expression by binding of FoxO1 to its promoter and subsequently inhibited Trx activity in human aortic endothelial cells [63]. These effects were Trx system-mediated reduction of oxidized cysteine groups on proteins through an interaction with the redox-active center of Trx and activated FoxO1 pathway.
On the other hand, FoxO1 may also protect against oxidative stress in cardiomyocytes by promoting the expression of antioxidant enzymes such as catalase (CAT) and manganese superoxide dismutase (MnSOD) via Yes-associated protein (YAP) pathways to neutralize ROS [64]. STZ-induced diabetic rats with myocardial metabolism and functional abnormalities showed oxidative stress by reduced activity of SOD, and elevated malondialdehyde [MDA] levels [52]. Curcumin treatment in these rats rescued the activity of SOD by restoring Sirt1-FoxO1 signaling, resulting in reduced ROS and alleviation of DbCM. Moreover, Exenatide, a glucagon-like peptide-1 (GLP-1) receptor agonist, attenuated ROS production through increases in expression of MnSOD and catalase in cardiomyocytes of HFD-fed T2D mice and STZ-induced T1D mice [65]. These protective actions might be mediated through Sirt1-FoxO1 pathways, as the cardioprotective effects of Exendin-4 against ischemia/reperfusion (I/R) injury in male rats involves upregulated activity Sirt1-FoxO1 pathways and associated MnSOD production [66]. Thus, in varying microenvironments such as the level of stress in various cell types of diabetic myocardium or ROS-mediated signaling activation, FoxO1 may play a destructive rather than protective role during oxidative stress regulations [67]. In diabetic myocardium, conditions like hyperglycemia, insulin resistance, and metabolic disturbances such as elevated serum glucose or lipids can induce FoxO1 expression, shifting its function from antioxidant to prooxidant.
FoxO1 in diabetic cardiomyopathy-associated myocardial cell death
Apart from its roles in energy metabolism and oxidative stress, FoxO1 also has substantial roles in myocardial cell death via apoptosis and autophagy during diabetes [68]. In diabetic myocardium, upregulated FoxO1 activity stimulates the expression of various proapoptotic regulators such as B-cell lymphoma 2 (Bcl2)-associated agonist of cell death (BAD), Bcl-2 Interacting Mediator (BIM), Puma, and caspases [46, 48]. Puthanveetil et al. demonstrated that FoxO1 regulates BAD via up-regulation of protein phosphatase 2A (PP2A) in the diabetic myocardium [48]. However, in cardiomyocytes, overexpression of the wild-type or constitutively active form of FoxO1 has been associated with inhibition of the PP2A/B activity and attenuation of insulin signaling [69]. This divergence likely stems from FoxO1/CD36-mediated lipid buildup in diabetic cardiomyocytes, which may reactivate PP2A and trigger BAD activation, similar to how ceramides stimulate PP2A during arterial dysfunction in obese mice [70]. It is noteworthy that, myocardial apoptosis may not be regulated by FoxO1 in all available pre-clinical models of DbCM. Our recent study demonstrated the attenuation of diastolic dysfunction and altered myocardial metabolism but no effect on apoptosis by pharmacological or genetic inhibition of FoxO1 in T2D mice [46]. However, FoxO1 inhibition by AS1842856 in T1D male Sprague Dawley rats mitigated the apoptosis, evident by a reduction in cleaved caspase 3 expression and tunnel staining [47]. Similarly, curcumin was shown to alleviate apoptosis in cardiomyocytes which was associated with inhibition of FoxO1 acetylation and modulation of Sirt1-FoxO1 signaling in STZ-induced T2D rats [52]. Moreover, the opening of mitochondrial ATP-sensitive potassium (mitoKATP) channels by diazoxide was found to improve cardiac function and attenuate cardiomyocyte apoptosis in db/db mice [53]. The protective effect of diazoxide was associated with a reduction in AKT-FoxO1 signaling and the activity of caspase 3 in cardiomyocytes.
While the significance of autophagy in DbCM is still subject to debate, FoxO1 has been implicated in its regulation. In starvation, FoxO1 can activate the expression of autophagic genes such as autophagy-related protein 12 (Atg12) and γ-aminobutyric acid receptor-associated protein-like 1 (Gabarapl1) in cardiomyocytes [71]. Similarly, glucose-deprived cultured cardiomyocytes showed increased autophagic flux accompanied by Sirt1-associated FoxO1 deacetylation and a decreased expression of ubiquitin-binding protein p62 [72]. Contrarily, acetylated FoxO1 has been shown to upregulate autophagy in a transcription-independent manner by interacting with Atg7 in the cytosol of cancer cells [73]. FoxO1 also plays an essential role in the regression of cardiac hypertrophy via upregulating autophagy during mechanical unloading by reversal of transverse aortic constriction (TAC) in mice [74]. Similarly, FoxO1 contributes to exercise-induced physiological hypertrophy by regulating autophagy markers independent of the PI3K-AKT signaling [75]. Moreover, cardiac-specific overexpression of FoxO1 in transgenic mice exhibited a decrease in the size of hearts and upregulation of autophagy. Concurrently, it has been demonstrated that the cardioprotective effect of angiotensin (Ang) IV in T1D mice was through suppression of FoxO1-induced excessive autophagy [54]. The protective effects of Ang IV were completely blocked by over-expression of FoxO1, which was reversed by the additional administration of AS1842856. However, resveratrol has been shown to protect against DbCM by restoring autophagic flux [55]. The effect was achieved through the upregulation of FoxO1-mediated transcription of rat sarcoma virus-related protein (Rab)7, a small GTP-binding protein that mediates late autophagosome-lysosome fusion. Thus, the enhancement of FoxO1 activity contributes to dysregulated apoptosis and autophagy in diabetes, and targeting these perturbations could alleviate the progression of DbCM.
Discussion
Taken together, FoxO1 dysregulations could exacerbate damages in myocardial cellular processes, accelerating the development of diastolic dysfunction during DbCM, a major complication in people with diabetes. Metabolic alterations, oxidative stress, and cell death are implicated in both the progression of DbCM and the regulatory processes involving FoxO1. Enhanced FoxO1 expression and activity appear to promote alteration in myocardial glucose and fatty acid metabolism, oxidative stress, and cell death in DbCM. Notably, the above-discussed findings are mainly based on animal models of T1D or T2D and clinical applications of FoxO1 signaling in cardiac injury in DbCM are still unknown. Moreover, the interplay between different molecular mediators of DbCM and their regulation by FoxO1 in pre-clinical models is largely unknown to predict a translational aspect of these findings. Thus, we currently lack enough information on whether FoxO1 or its pathways could be a therapeutic target during DbCM in people with diabetes. A promising approach could be the optimization of cardiac energy metabolism, though an improved understanding of how FoxO1-mediated modulations of myocardial energy metabolism, oxidative stress, cell death, and its interplay regulate diastole may direct us to better molecular targets for future drug development.
Author contributions
All authors listed have made a substantial, direct, and intellectual contribution to the work and approved it for publication.
Funding
The authors declare that financial support was received for the research, authorship, and/or publication of this article. The work is supported by the Faculty of Pharmacy and Pharmaceutical Sciences, University of Alberta.
Acknowledgments
The figure in this review article was created with BioRender.com.
Conflict of interest
The authors declare that the research was conducted in the absence of any commercial or financial relationships that could be construed as a potential conflict of interest.
References
1. Saeedi, P, Petersohn, I, Salpea, P, Malanda, B, Karuranga, S, Unwin, N, et al. Global and regional diabetes prevalence estimates for 2019 and projections for 2030 and 2045: results from the International Diabetes Federation Diabetes Atlas, 9(th) edition. Diabetes Res Clin Pract (2019) 157:107843. doi:10.1016/j.diabres.2019.107843
2. Scherer, PE, and Hill, JA. Obesity, diabetes, and cardiovascular diseases. Circ Res (2016) 118(11):1703–5. doi:10.1161/circresaha.116.308999
3. Kannel, WB, and McGee, DL. Diabetes and cardiovascular disease. The Framingham study. J Am Med Assoc (1979) 241(19):2035–8. doi:10.1001/jama.241.19.2035
4. Kannel, WB, and McGee, DL. Diabetes and glucose tolerance as risk factors for cardiovascular disease: the Framingham study. Diabetes Care (1979) 2(2):120–6. doi:10.2337/diacare.2.2.120
5. Ritchie, RH, and Abel, ED. Basic mechanisms of diabetic heart disease. Circ Res (2020) 126(11):1501–25. doi:10.1161/circresaha.120.315913
6. Heather, LC, Gopal, K, Srnic, N, and Ussher, JR. Redefining diabetic cardiomyopathy: perturbations in substrate metabolism at the heart of its pathology. Diabetes (2024) 73:659–70. doi:10.2337/dbi23-0019
7. van der Horst, A, and Burgering, BMT. Stressing the role of FoxO proteins in lifespan and disease. Nat Rev Mol Cel Biol (2007) 8(6):440–50. doi:10.1038/nrm2190
8. Ronnebaum, SM, and Patterson, C. The FoxO family in cardiac function and dysfunction. Annu Rev Physiol (2010) 72(1):81–94. doi:10.1146/annurev-physiol-021909-135931
9. Battiprolu, PK, Hojayev, B, Jiang, N, Wang, ZV, Luo, X, Iglewski, M, et al. Metabolic stress-induced activation of FoxO1 triggers diabetic cardiomyopathy in mice. J Clin Invest (2012) 122(3):1109–18. doi:10.1172/jci60329
10. Kandula, V, Kosuru, R, Li, H, Yan, D, Zhu, Q, Lian, Q, et al. Forkhead box transcription factor 1: role in the pathogenesis of diabetic cardiomyopathy. Cardiovasc Diabetology (2016) 15(1):44. doi:10.1186/s12933-016-0361-1
11. Rubler, S, Dlugash, J, Yuceoglu, YZ, Kumral, T, Branwood, AW, and Grishman, A. New type of cardiomyopathy associated with diabetic glomerulosclerosis. The Am J Cardiol (1972) 30(6):595–602. doi:10.1016/0002-9149(72)90595-4
12. Ho, CY, and Solomon, SD. A clinician’s guide to tissue Doppler imaging. Circulation (2006) 113(10):e396–8. doi:10.1161/circulationaha.105.579268
13. Lindsey, ML, Kassiri, Z, Virag, JAI, de Castro Bras, LE, and Scherrer-Crosbie, M. Guidelines for measuring cardiac physiology in mice. Am J Physiology-Heart Circulatory Physiol (2018) 314(4):H733–H752. doi:10.1152/ajpheart.00339.2017
14. Poirier, P, Bogaty, P, Garneau, C, Marois, L, and Dumesnil, JG. Diastolic dysfunction in normotensive men with well-controlled type 2 diabetes: importance of maneuvers in echocardiographic screening for preclinical diabetic cardiomyopathy. Diabetes Care (2001) 24(1):5–10. doi:10.2337/diacare.24.1.5
15. Fang, ZY, Schull-Meade, R, Leano, R, Mottram, PM, Prins, JB, and Marwick, TH. Screening for heart disease in diabetic subjects. Am Heart J (2005) 149(2):349–54. doi:10.1016/j.ahj.2004.06.021
16. Yazici, M, Ozdemir, K, Gonen, MS, Kayrak, M, Ulgen, MS, Duzenli, MA, et al. Is there any relationship between metabolic parameters and left ventricular functions in type 2 diabetic patients without evident heart disease? Echocardiography (2008) 25(7):675–82. doi:10.1111/j.1540-8175.2008.00690.x
17. Boyer, JK, Thanigaraj, S, Schechtman, KB, and Perez, JE. Prevalence of ventricular diastolic dysfunction in asymptomatic, normotensive patients with diabetes mellitus. The Am J Cardiol (2004) 93(7):870–5. doi:10.1016/j.amjcard.2003.12.026
18. Echouffo-Tcheugui, JB, Xu, H, DeVore, AD, Schulte, PJ, Butler, J, Yancy, CW, et al. Temporal trends and factors associated with diabetes mellitus among patients hospitalized with heart failure: findings from Get with the Guidelines-Heart Failure registry. Am Heart J (2016) 182:9–20. doi:10.1016/j.ahj.2016.07.025
19. Jia, G, Hill, MA, and Sowers, JR. Diabetic cardiomyopathy: an update of mechanisms contributing to this clinical entity. Circ Res (2018) 122(4):624–38. doi:10.1161/circresaha.117.311586
20. Heather, LC, Hafstad, AD, Halade, GV, Harmancey, R, Mellor, KM, Mishra, PK, et al. Guidelines on models of diabetic heart disease. Am J Physiology-Heart Circulatory Physiol (2022) 323(1):H176–H200. doi:10.1152/ajpheart.00058.2022
21. Battiprolu, PK, Gillette, TG, Wang, ZV, Lavandero, S, and Hill, JA. Diabetic cardiomyopathy: mechanisms and therapeutic targets. Drug Discov Today Dis Mech (2010) 7(2):e135–e143. doi:10.1016/j.ddmec.2010.08.001
22. Zlobine, I, Gopal, K, and Ussher, JR. Lipotoxicity in obesity and diabetes-related cardiac dysfunction. Biochim Biophys Acta (Bba) - Mol Cel Biol Lipids (2016) 1861(10):1555–68. doi:10.1016/j.bbalip.2016.02.011
23. Boudina, S, Bugger, H, Sena, S, O'Neill, BT, Zaha, VG, Ilkun, O, et al. Contribution of impaired myocardial insulin signaling to mitochondrial dysfunction and oxidative stress in the heart. Circulation (2009) 119(9):1272–83. doi:10.1161/circulationaha.108.792101
24. Tsushima, K, Bugger, H, Wende, AR, Soto, J, Jenson, GA, Tor, AR, et al. Mitochondrial reactive oxygen species in lipotoxic hearts induce post-translational modifications of AKAP121, DRP1, and OPA1 that promote mitochondrial fission. Circ Res (2018) 122(1):58–73. doi:10.1161/circresaha.117.311307
25. Weigel, D, Jürgens, G, Küttner, F, Seifert, E, and Jäckle, H. The homeotic gene fork head encodes a nuclear protein and is expressed in the terminal regions of the Drosophila embryo. Cell (1989) 57(4):645–58. doi:10.1016/0092-8674(89)90133-5
26. Weigel, D, and Jäckle, H. The fork head domain: a novel DNA binding motif of eukaryotic transcription factors? Cell (1990) 63(3):455–6. doi:10.1016/0092-8674(90)90439-l
27. Ogg, S, Paradis, S, Gottlieb, S, Patterson, GI, Lee, L, Tissenbaum, HA, et al. The Fork head transcription factor DAF-16 transduces insulin-like metabolic and longevity signals in C. elegans. Nature (1997) 389(6654):994–9. doi:10.1038/40194
28. Galili, N, Davis, RJ, Fredericks, WJ, Mukhopadhyay, S, Rauscher, FJ, Emanuel, BS, et al. Fusion of a fork head domain gene to PAX3 in the solid tumour alveolar rhabdomyosarcoma. Nat Genet (1993) 5(3):230–5. doi:10.1038/ng1193-230
29. Monsalve, M, and Olmos, Y. The complex biology of FOXO. Curr Drug Targets (2011) 12(9):1322–50. doi:10.2174/138945011796150307
30. Hannenhalli, S, and Kaestner, KH. The evolution of Fox genes and their role in development and disease. Nat Rev Genet (2009) 10(4):233–40. doi:10.1038/nrg2523
31. Kim, DH, Perdomo, G, Zhang, T, Slusher, S, Lee, S, Phillips, BE, et al. FoxO6 integrates insulin signaling with gluconeogenesis in the liver. Diabetes (2011) 60(11):2763–74. doi:10.2337/db11-0548
32. Obsil, T, and Obsilova, V. Structure/function relationships underlying regulation of FOXO transcription factors. Oncogene (2008) 27(16):2263–75. doi:10.1038/onc.2008.20
33. Santos, BF, Grenho, I, Martel, PJ, Ferreira, BI, and Link, W. FOXO family isoforms. Cel Death and Dis (2023) 14(10):702. doi:10.1038/s41419-023-06177-1
34. Eijkelenboom, A, and Burgering, BMT. FOXOs: signalling integrators for homeostasis maintenance. Nat Rev Mol Cel Biol (2013) 14(2):83–97. doi:10.1038/nrm3507
35. Zhang, X, Tang, N, Hadden, TJ, and Rishi, AK. Akt, FoxO and regulation of apoptosis. Biochim Biophys Acta (Bba) - Mol Cel Res (2011) 1813(11):1978–86. doi:10.1016/j.bbamcr.2011.03.010
36. Puthanveetil, P, Wan, A, and Rodrigues, B. FoxO1 is crucial for sustaining cardiomyocyte metabolism and cell survival. Cardiovasc Res (2013) 97(3):393–403. doi:10.1093/cvr/cvs426
37. Klotz, L-O, Sánchez-Ramos, C, Prieto-Arroyo, I, Urbánek, P, Steinbrenner, H, and Monsalve, M. Redox regulation of FoxO transcription factors. Redox Biol (2015) 6:51–72. doi:10.1016/j.redox.2015.06.019
38. Han, R, Huang, H, Xia, W, Liu, J, Luo, H, Tang, J, et al. Perspectives for Forkhead box transcription factors in diabetic cardiomyopathy: their therapeutic potential and possible effects of salvianolic acids. Front Cardiovasc Med (2022) 9:951597. doi:10.3389/fcvm.2022.951597
39. Qi, Y, Zhu, Q, Zhang, K, Thomas, C, Wu, Y, Kumar, R, et al. Activation of foxo1 by insulin resistance promotes cardiac dysfunction and β–myosin heavy chain gene expression. Circ Heart Fail (2015) 8(1):198–208. doi:10.1161/circheartfailure.114.001457
40. Ni, YG, Berenji, K, Wang, N, Oh, M, Sachan, N, Dey, A, et al. Foxo transcription factors blunt cardiac hypertrophy by inhibiting calcineurin signaling. Circulation (2006) 114(11):1159–68. doi:10.1161/circulationaha.106.637124
41. Cai, B, Wang, N, Mao, W, You, T, Lu, Y, Li, X, et al. Deletion of FoxO1 leads to shortening of QRS by increasing Na+ channel activity through enhanced expression of both cardiac NaV1.5 and β3 subunit. J Mol Cell Cardiol (2014) 74:297–306. doi:10.1016/j.yjmcc.2014.06.006
42. Hannenhalli, S, Putt, ME, Gilmore, JM, Wang, J, Parmacek, MS, Epstein, JA, et al. Transcriptional genomics associates FOX transcription factors with human heart failure. Circulation (2006) 114(12):1269–76. doi:10.1161/circulationaha.106.632430
43. Auguste, G, Gurha, P, Lombardi, R, Coarfa, C, Willerson, JT, and Marian, AJ. Suppression of activated FOXO transcription factors in the heart prolongs survival in a mouse model of laminopathies. Circ Res (2018) 122(5):678–92. doi:10.1161/circresaha.117.312052
44. Bugger, H, and Abel, ED. Molecular mechanisms of diabetic cardiomyopathy. Diabetologia (2014) 57(4):660–71. doi:10.1007/s00125-014-3171-6
45. Gopal, K, Saleme, B, Al Batran, R, Aburasayn, H, Eshreif, A, Ho, KL, et al. FoxO1 regulates myocardial glucose oxidation rates via transcriptional control of pyruvate dehydrogenase kinase 4 expression. Am J Physiology-Heart Circulatory Physiol (2017) 313(3):H479–H490. doi:10.1152/ajpheart.00191.2017
46. Gopal, K, Al Batran, R, Altamimi, TR, Greenwell, AA, Saed, CT, Tabatabaei Dakhili, SA, et al. FoxO1 inhibition alleviates type 2 diabetes-related diastolic dysfunction by increasing myocardial pyruvate dehydrogenase activity. Cel Rep (2021) 35(1):108935. doi:10.1016/j.celrep.2021.108935
47. Yan, D, Cai, Y, Luo, J, Liu, J, Li, X, Ying, F, et al. FOXO1 contributes to diabetic cardiomyopathy via inducing imbalanced oxidative metabolism in type 1 diabetes. J Cell Mol Med (2020) 24:7850–61. doi:10.1111/jcmm.15418
48. Puthanveetil, P, Wang, Y, Zhang, D, Wang, F, Kim, MS, Innis, S, et al. Cardiac triglyceride accumulation following acute lipid excess occurs through activation of a FoxO1–iNOS–CD36 pathway. Free Radic Biol Med (2011) 51(2):352–63. doi:10.1016/j.freeradbiomed.2011.04.009
49. Pham, TK, Nguyen, THT, Yi, JM, Kim, GS, Yun, HR, Kim, HK, et al. Evogliptin, a DPP-4 inhibitor, prevents diabetic cardiomyopathy by alleviating cardiac lipotoxicity in db/db mice. Exp and Mol Med (2023) 55(4):767–78. doi:10.1038/s12276-023-00958-6
50. Ying, F, Liu, H, Ching Tang, EH, Lakhani, I, Liu, N, Xia, Z, et al. Prostaglandin E receptor subtype 4 protects against diabetic cardiomyopathy by modulating cardiac fatty acid metabolism via FOXO1/CD36 signalling. Biochem Biophysical Res Commun (2021) 548:196–203. doi:10.1016/j.bbrc.2021.01.038
51. Kyriazis, ID, Hoffman, M, Gaignebet, L, Lucchese, AM, Markopoulou, E, Palioura, D, et al. KLF5 is induced by FOXO1 and causes oxidative stress and diabetic cardiomyopathy. Circ Res (2021) 128(3):335–57. doi:10.1161/circresaha.120.316738
52. Ren, B, Zhang, Y, Liu, S, Cheng, X, Yang, X, Cui, X, et al. Curcumin alleviates oxidative stress and inhibits apoptosis in diabetic cardiomyopathy via Sirt1-Foxo1 and PI3K-Akt signalling pathways. J Cell Mol Med (2020) 24(21):12355–67. doi:10.1111/jcmm.15725
53. Duan, P, Wang, J, Li, Y, Wei, S, Su, F, Zhang, S, et al. Opening of mitoKATP improves cardiac function and inhibits apoptosis via the AKT-Foxo1 signaling pathway in diabetic cardiomyopathy. Int J Mol Med (2018) 42:2709–19. doi:10.3892/ijmm.2018.3832
54. Zhang, M, Sui, W, Xing, Y, Cheng, J, Cheng, C, Xue, F, et al. Angiotensin IV attenuates diabetic cardiomyopathy via suppressing FoxO1-induced excessive autophagy, apoptosis and fibrosis. Theranostics (2021) 11(18):8624–39. doi:10.7150/thno.48561
55. Wang, B, Yang, Q, Sun, Y, Xing, Y, Wang, Y, Lu, X, et al. Resveratrol-enhanced autophagic flux ameliorates myocardial oxidative stress injury in diabetic mice. J Cell Mol Med (2014) 18(8):1599–611. doi:10.1111/jcmm.12312
56. Mazumder, PK, O'Neill, BT, Roberts, MW, Buchanan, J, Yun, UJ, Cooksey, RC, et al. Impaired cardiac efficiency and increased fatty acid oxidation in insulin-resistant ob/ob mouse hearts. Diabetes (2004) 53(9):2366–74. doi:10.2337/diabetes.53.9.2366
57. Karwi, QG, Uddin, GM, Ho, KL, and Lopaschuk, GD. Loss of metabolic flexibility in the failing heart. Front Cardiovasc Med (2018) 5:68. doi:10.3389/fcvm.2018.00068
58. Chambers, KT, Leone, TC, Sambandam, N, Kovacs, A, Wagg, CS, Lopaschuk, GD, et al. Chronic inhibition of pyruvate dehydrogenase in heart triggers an adaptive metabolic response. J Biol Chem (2011) 286(13):11155–62. doi:10.1074/jbc.m110.217349
59. Rijzewijk, LJ, van der Meer, RW, Smit, JW, Diamant, M, Bax, JJ, Hammer, S, et al. Myocardial steatosis is an independent predictor of diastolic dysfunction in type 2 diabetes mellitus. J Am Coll Cardiol (2008) 52(22):1793–9. doi:10.1016/j.jacc.2008.07.062
60. Luiken, JJ, Dyck, DJ, Han, XX, Tandon, NN, Arumugam, Y, Glatz, JF, et al. Insulin induces the translocation of the fatty acid transporter FAT/CD36 to the plasma membrane. Am J Physiology-Endocrinology Metab (2002) 282(2):E491–5. doi:10.1152/ajpendo.00419.2001
61. Faria, A, and Persaud, SJ. Cardiac oxidative stress in diabetes: mechanisms and therapeutic potential. Pharmacol and Ther (2017) 172:50–62. doi:10.1016/j.pharmthera.2016.11.013
62. Coughlan, MT, Thorburn, DR, Penfold, SA, Laskowski, A, Harcourt, BE, Sourris, KC, et al. RAGE-induced cytosolic ROS promote mitochondrial superoxide generation in diabetes. J Am Soc Nephrol (2009) 20(4):742–52. doi:10.1681/asn.2008050514
63. Li, X, Rong, Y, Zhang, M, Wang, XL, LeMaire, SA, Coselli, JS, et al. Up-regulation of thioredoxin interacting protein (Txnip) by p38 MAPK and FOXO1 contributes to the impaired thioredoxin activity and increased ROS in glucose-treated endothelial cells. Biochem Biophysical Res Commun (2009) 381(4):660–5. doi:10.1016/j.bbrc.2009.02.132
64. Shao, D, Zhai, P, Del Re, DP, Sciarretta, S, Yabuta, N, Nojima, H, et al. A functional interaction between Hippo-YAP signalling and FoxO1 mediates the oxidative stress response. Nat Commun (2014) 5(1):3315. doi:10.1038/ncomms4315
65. Ding, W, Chang, W-g, Guo, X-c, Liu, Y, Xiao, D-d, Ding, D, et al. Exenatide protects against cardiac dysfunction by attenuating oxidative stress in the diabetic mouse heart. Front Endocrinol (2019) 10:202. doi:10.3389/fendo.2019.00202
66. Eid, RA, Bin-Meferij, MM, El-kott, AF, Eleawa, SM, Zaki, MSA, Al-Shraim, M, et al. Exendin-4 protects against myocardial ischemia-reperfusion injury by upregulation of SIRT1 and SIRT3 and activation of AMPK. J Cardiovasc Translational Res (2020) 14(4):619–35. doi:10.1007/s12265-020-09984-5
67. Ponugoti, B, Dong, G, and Graves, DT. Role of forkhead transcription factors in diabetes-induced oxidative stress. Exp Diabetes Res (2012) 2012:1–7. doi:10.1155/2012/939751
68. Gross, DN, van den Heuvel, APJ, and Birnbaum, MJ. The role of FoxO in the regulation of metabolism. Oncogene (2008) 27(16):2320–36. doi:10.1038/onc.2008.25
69. Ni, YG, Wang, N, Cao, DJ, Sachan, N, Morris, DJ, Gerard, RD, et al. FoxO transcription factors activate Akt and attenuate insulin signaling in heart by inhibiting protein phosphatases. Proc Natl Acad Sci U S A. (2007) 104(51):20517–22. doi:10.1073/pnas.0610290104
70. Bharath, LP, Ruan, T, Li, Y, Ravindran, A, Wan, X, Nhan, JK, et al. Ceramide-initiated protein phosphatase 2A activation contributes to arterial dysfunction in vivo. Diabetes (2015) 64(11):3914–26. doi:10.2337/db15-0244
71. Sengupta, A, Molkentin, JD, and Yutzey, KE. FoxO transcription factors promote autophagy in cardiomyocytes. J Biol Chem (2009) 284(41):28319–31. doi:10.1074/jbc.m109.024406
72. Hariharan, N, Maejima, Y, Nakae, J, Paik, J, Depinho, RA, and Sadoshima, J. Deacetylation of FoxO by Sirt1 plays an essential role in mediating starvation-induced autophagy in cardiac myocytes. Circ Res (2010) 107(12):1470–82. doi:10.1161/circresaha.110.227371
73. Zhao, Y, Yang, J, Liao, W, Liu, X, Zhang, H, Wang, S, et al. Cytosolic FoxO1 is essential for the induction of autophagy and tumour suppressor activity. Nat Cel Biol (2010) 12(7):665–75. doi:10.1038/ncb2069
74. Hariharan, N, Ikeda, Y, Hong, C, Alcendor, RR, Usui, S, Gao, S, et al. Autophagy plays an essential role in mediating regression of hypertrophy during unloading of the heart. PLoS ONE (2013) 8(1):e51632. doi:10.1371/journal.pone.0051632
Keywords: FoxO1, diabetic cardiomyopathy, energy metabolism, oxidative stress, cell death
Citation: Shafaati T and Gopal K (2024) Forkhead box O1 transcription factor; a therapeutic target for diabetic cardiomyopathy. J. Pharm. Pharm. Sci 27:13193. doi: 10.3389/jpps.2024.13193
Received: 26 April 2024; Accepted: 31 July 2024;
Published: 14 August 2024.
Edited by:
Sherif Hanafy Mahmoud, University of Alberta, CanadaCopyright © 2024 Shafaati and Gopal. This is an open-access article distributed under the terms of the Creative Commons Attribution License (CC BY). The use, distribution or reproduction in other forums is permitted, provided the original author(s) and the copyright owner(s) are credited and that the original publication in this journal is cited, in accordance with accepted academic practice. No use, distribution or reproduction is permitted which does not comply with these terms.
*Correspondence: Keshav Gopal, Z29wYWxAdWFsYmVydGEuY2E=
†ORCID: Tanin Shafaati, https://orcid.org/0009-0000-9643-9429