- 1Lunenfeld-Tanenbaum Research Institute, Mount Sinai Hospital, Toronto, ON, Canada
- 2Faculty of Pharmaceutical Sciences, University of British Columbia, Vancouver, BC, Canada
Worldwide, the prevalence of obesity and diabetes have increased, with heart disease being their leading cause of death. Traditionally, the management of obesity and diabetes has focused mainly on weight reduction and controlling high blood glucose. Unfortunately, despite these efforts, poor medication management predisposes these patients to heart failure. One instigator for the development of heart failure is how cardiac tissue utilizes different sources of fuel for energy. In this regard, the heart switches from using various substrates, to predominantly using fatty acids (FA). This transformation to using FA as an exclusive source of energy is helpful in the initial stages of the disease. However, over the progression of diabetes this has grave end results. This is because toxic by-products are produced by overuse of FA, which weaken heart function (heart disease). Lipoprotein lipase (LPL) is responsible for regulating FA delivery to the heart, and its function during diabetes has not been completely revealed. In this review, the mechanisms by which LPL regulates fuel utilization by the heart in control conditions and following diabetes will be discussed in an attempt to identify new targets for therapeutic intervention. Currently, as treatment options to directly target diabetic heart disease are scarce, research on LPL may assist in drug development that exclusively targets fuel utilization by the heart and lipid accumulation in macrophages to help delay, prevent, or treat cardiac failure, and provide long-term management of this condition during diabetes.
Introduction
Continuous beating is a distinctive feature of the heart. As such, cardiomyocytes, which are responsible for this heart contraction, have a high requirement for energy and acquire it from several sources like fatty acids (FA) and glucose in addition to amino acids, lactate and ketones. Among these, the majority of ATP produced in the heart is made from glucose and FA through mitochondrial metabolism, with FA being the favored substrate. The heart is unable to synthesize FA and obtains it from other sources. These include a) release from adipose tissue triglyceride (TG) stores, b) endogenous TG within lipid droplets in the heart, and c) breakdown of circulating TG-rich lipoproteins to FA by lipoprotein lipase (LPL) positioned at the endothelial cell (EC) surface of the coronary lumen. Of these, LPL-mediated breakdown of lipoproteins is suggested to be a major source of FA for cardiac energy generation. This review will cover the participation of LPL in FA delivery to the heart (for generation of energy) and adipose tissue (for storage as TG), and the consequences of its tissue mismanagement following diabetes. Specifically, we will focus on LPL function and dysfunction, and its contribution towards the development of both atherosclerosis and cardiomyopathy. It is hoped that by understanding LPL regulation and modification following diabetes, we can advance the clinical management of diabetic heart disease as it relates to FA metabolism.
Cardiac lipoprotein lipase—preamble
The breakdown of circulating TG in lipoproteins by LPL occurs in the vascular lumen. However, endothelial cells (EC) that line the lumen are incapable of producing LPL [1–3]. Using the heart (where LPL can be examined at different sites), it has been documented that this enzyme is made in cardiomyocytes before it is moved to the coronary lumen. Thus, immunogold labeling of LPL confirmed that in the heart, about 80% of LPL is present in cardiomyocytes, 18% is located at the capillary EC, and the remaining amount is located in the interstitial space (Figure 1) [4]. Related to its synthesis in cardiomyocytes, LPL has been reported to be produced as a monomer (inactive) in the endoplasmic reticulum. Enzyme activation follows dimerization, with subsequent cellular secretion [5, 6]. Recent evidence has suggested that monomeric LPL also shows enzyme activity [7]. Following its synthesis, there are two proteins that are important for LPL maturation (folding) and transport; lipase maturation factor and suppressor of lin-12-like protein 1 [8]. Intracellular vesicles then store LPL bound to syndecan-1 [9]. Vesicular movement to the cell surface permits LPL secretion onto heparan sulphate proteoglycan (HSPG) binding sites on the outer surface of cardiomyocytes [10]. Attachment of the positively charged LPL to HSPG is made possible by ionic binding to the heparan sulfate (HS) side chains that is negatively charged. Location of LPL at this site serves as a rapidly accessible pool for the heart when it requires energy in the form of FAs [11, 12]. For its onward movement from the myocyte cell surface to the coronary lumen, LPL is released from HSPG, crosses the interstitial space and binds to glycosylphosphatidylinositol-anchored high-density lipoprotein-binding protein 1 (GPIHBP1) on the basolateral side of EC [13]. GPIHBP1 functions as a transporter, moving LPL from the basolateral side of EC to the capillary lumen [14–16]. At the lumen, GPIHBP1 can bind LPL and circulating lipoproteins to promote TG hydrolysis and supply FA to the underlying cardiomyocytes for energy production [16–18] (see detailed review [19]). Interestingly, the action of LPL on chylomicron (lipoprotein produced mainly in the gut) clearance is likely more substantial than its effect on VLDL (lipoprotein synthesised mainly in the liver) hydrolysis. Compared to VLDL, chylomicrons are larger, contain a greater amount of TG and have a better chance to interact with coronary LPL [20]. In addition, LPL breakdown of VLDL yields FA that require a FA transporter, CD36, for movement into cells, whereas its breakdown of chylomicrons produces a higher amount of FA that use a passive flip-flop and/or CD36-independent transport mechanisms [21]. A more recent study produced lipoproteins by administering radiolabelled 2H-FA by gastric gavage. Following isolation and i.v. injection of the lipoproteins (d = 1.006 g/mL), a rapid (as quickly as 2 min) accumulation of radiolabelled FA within the cytosol and mitochondria of cardiomyocytes was observed, indicating that EC did not serve as a storehouse for FA as they traveled from the vascular lumen to the underlying cardiomyocytes [22]. Additionally, as a deficiency in CD36 did not modify the passage of lipoprotein FA into cardiomyocytes, the authors concluded that LPL derived FA can be taken up quickly by cardiomyocytes without the need for FA carriers [22]. Finally, when LPL-derived FA are compared to non-esterified fatty acid (NEFA) derived from adipose tissue, our data suggest that following fasting, NEFA plays a central role in energy generation [23], whereas LPL action also provides for lipid accumulation in the heart [24].
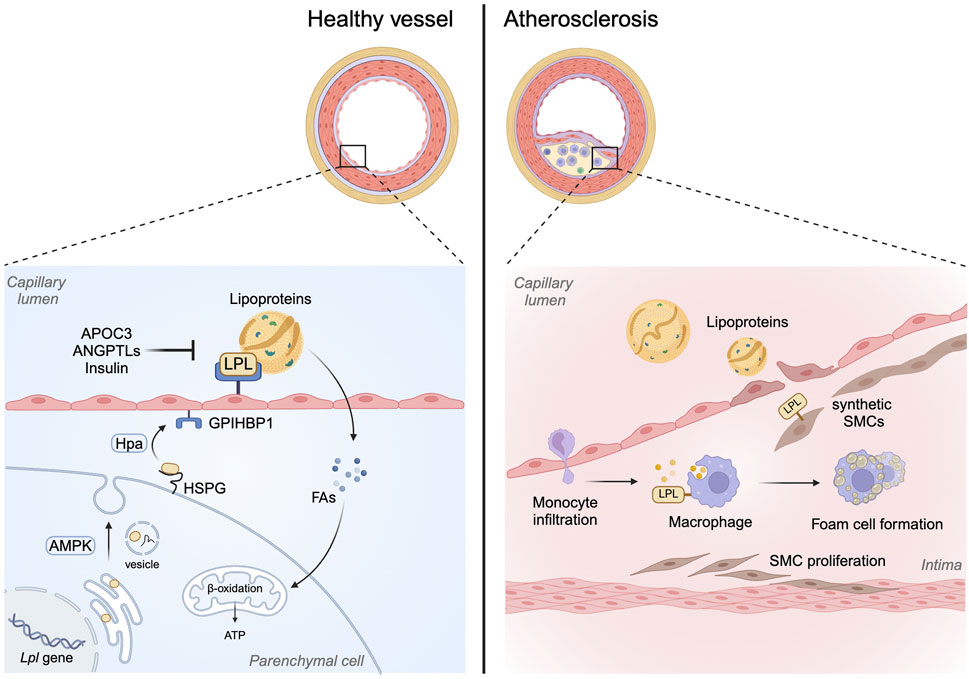
Figure 1. Role of LPL beyond its lipolytic action. LPL has traditionally been known to facilitate lipoprotein hydrolysis to release FA. This action of LPL normally occurs at the endothelial lining of the vasculature following the translocation of LPL from subjacent parenchymal cells to the apical side of endothelial cells. Posttranslationally, LPL activity can be regulated by a number of mechanisms including APOC3, ANGPTLs and insulin. On the other hand, in macrophages, the action of LPL is mainly to promote remnant cholesterol uptake, foam cell formation and plaque development in arteries. This bridging function of LPL in SMCs, especially synthetic cells that make up the plaque, may also contribute towards lipoprotein uptake and foam cell formation.
Posttranslational processes that regulate cardiac LPL
Of the multiple substrates that the heart can use as an energy source, FA is the preferred fuel. As such, intrinsic mechanisms have been developed by the heart to regulate delivery of this substrate, with LPL being a major player. Several mediators are present which modulate cardiac LPL. These include:
AMP-activated protein kinase (AMPK)
Upon a reduction in energy, AMPK is activated to stimulate energy producing pathways and turn off energy consuming pathways to restore the ATP/ADP ratio. Thus, AMPK is known to inhibit acetyl-CoA carboxylase, lower malonyl-CoA and increase the activity of carnitine palmitoyltransferase-1 to facilitate FA uptake and oxidation in the mitochondria [25, 26]. Stimulation of AMPK is also known to modulate FA uptake via CD36 [27]. Our laboratory has extensively published on the role of AMPK in LPL translocation to the vascular lumen [28]. Specifically, we reported that AMPK plays a role in vesicular formation and subsequent movement of LPL along the actin cytoskeleton in cardiomyocyte through activation of heat shock protein 25 [2]. Thus, physiological and pathological processes that change AMPK are known to impact coronary LPL activity. For instance, following overnight fasting, AMPK is activated to increase coronary LPL, that guarantees FA delivery to meet the energy demand in this nutrient deficient condition [29]. Using streptozotocin (STZ) to induce moderate diabetes in rats, we reported that like fasting, acute hypoinsulinemia stimulated AMPK phosphorylation, and resulted in an augmented coronary LPL activity. This enabled the heart to switch its substrate utilization to exclusively using LPL-derived FA [10]. With a higher dose of STZ to induce severe diabetes, these animals developed both hyperglycemia and severe hyperlipidemia with increased circulating FA [30], that are known to inhibit AMPK activation [31]. In hearts from these animals, LPL activity was reduced and an unregulated uptake of NEFA resulted in cardiac lipotoxicity and dysfunction [30, 32]. Overall, our data suggested that activation of AMPK is a significant contributor towards LPL movement and subsequent FA utilization. Thus, agents that are capable of increasing cardiac LPL activity through the AMPK pathway may be useful for preventing NEFA uptake and lipotoxicity following diabetes.
Heparanase (Hpa)
Hpa is an endo-β-glucuronidase that is produced in EC as an inactive latent protein (HpaL). Following its synthesis, it is secreted to be taken up by HSPG and stored in lysosomes [33, 34]. At this location, enzyme processing results in a 50-kDa polypeptide that is significantly more active (HpaA) than HpaL [35, 36]. Both forms of Hpa are stored within the EC until secreted in response to various stimuli. Related to its physiological functioning, Hpa has roles in embryonic development, wound healing and hair growth [37]. Studies from our lab was the first to identify a novel role of Hpa in cardiac metabolism. In this regard, we described how Hpa released cardiomyocyte LPL for subsequent transfer to the vascular lumen for FA generation [38]. In people living with Type 2 diabetes, plasma and urine levels of Hpa are increased [39, 40]. In vitro studies using EC established that acute incubation of these cells with high glucose had a robust influence on Hpa secretion [41]. Using an animal model of STZ-induced diabetes, isolated hearts released significantly higher amounts of both forms of Hpa within the first 5 min, with HpaL secretion being greater than HpaA [42]. Related to HpaA, its heparan sulfate hydrolyzing ability would be capable of releasing myocyte surface-bound proteins including LPL. Intriguingly, enzymatically inactive HpaL is also able to initiate HSPG-clustering that activates p38 MAPK, Src, PI3K-Akt, and RhoA [38, 43–46]. These signalling pathways could then allow for replenishment of the cardiomyocyte pool of LPL that was released by HpaA. Overall, circulating Hpa has an important role in the communication between EC and cardiomyocytes to eventually supply FA to the heart. Intriguingly, unlike high glucose, when EC are exposed to increasing concentrations of palmitic acid, the nuclear content of Hpa was augmented [41]. Moreover, in recently published data from our lab, severe diabetes with concomitant hyperglycemia and hyperlipidemia reduced Hpa secretion from the isolated heart, a possible explanation for the lowered coronary LPL activity in these hearts [47]. Currently, whether manipulation of EC Hpa is capable of influencing cardiac metabolism following diabetes is unknown and should be investigated.
Heparan sulfate proteoglycans (HSPG)
To determine the contribution of HSPG to LPL transcytosis, LPL accumulation was determined following knock-out of GIPHBP1. In this condition, LPL in skeletal muscle and heart collected more at the basolateral side of EC as compared to the cardiomyocyte side suggesting that an HSPG gradient determines the direction of LPL flow from the underlying cardiomyocyte to the basolateral surface of EC [48]. At this location, collagen XVIII also acts as a reservoir for LPL [49].
GPIHBP1
LPL is expressed mainly in parenchymal cells like cardiomyocytes, whereas GPIHBP1 is located exclusively in capillary ECs. Interestingly, a comparable distribution of GPIHBP1 is described at both the luminal or abluminal sides of these cells [16, 50]. It should be noted that ECs that are part of large blood vessels, arterioles and venules do not display GPIHBP1 [16]. Regarding the binding of LPL to GPIHBP1, this occurs at a 1:1 ratio and with a higher affinity when compared to its binding to HSPG [51]. Structurally, as GPIHBP1 contains a GPI anchor, its release from the plasma membrane is achievable with phosphatidylinositol-specific phospholipase C that is known to digest this anchor [14]. It is the acidic domain of GPIHBP1 that can ionically attach LPL [52]. Given its defined role in the bidirectional translocation of LPL across the EC [16], and its ability to serve as a platform to promote lipoprotein-TG hydrolysis (it allows lipoproteins to stay bound/marginate to heart capillaries for several minutes [53, 54]), its absence in GPIHBP1 knockout mice causes robust hypertriglyceridemia even when these animals are fed a low-fat diet [16]. Similar effects are seen in patients with GPIHBP1 mutations [55]. More recent functions of GPIHBP1 include its ability to prevent the unfolding of LPL by angiopoietin-like protein 4 (ANGPTL4) [56–58]. Regarding its regulation, GPIHBP1 expression can be affected by fasting/refeeding [59]. Fasting augments cardiac GPIHBP1, and this effect can be overcome by refeeding [59]. Following diabetes, cardiac GPIHBP1 gene and protein expression also increase with an associated augmentation of coronary LPL activity [60]. Moreover, in vitro incubation of EC with high glucose also caused a rapid increase in GPIHBP1 mRNA and protein [50, 61]. Intriguingly, exposure of EC to HpaL or HpaA produced a significant increase in GPIHBP1 gene and protein [50]. Given that high glucose can stimulate the secretion of both forms of Hpa, this could be one mechanism by which the EC can increase GPIHBP1 to accelerate FA delivery to the cardiomyocytes after diabetes.
Angiopoietin-like proteins (ANGPTLs) regulation of LPL
ANGPTL 3, 4, and 8 are endogenous LPL antagonists. ANGPTL3 is exclusively expressed in the liver whereas ANGPTL4 and 8 are abundant in the liver, adipose tissue and muscle. One way by which fasting decreases LPL in the adipose tissue is that nutritional deprivation increases ANGPTL4 in adipose tissue. This is a positive effect as circulating TG are then diverted towards oxidative tissues for provision of energy [62].
Fatty acids (FA)
FA is known to affect LPL in multiple ways, including a) FA inhibition of LPL movement in the cardiomyocyte [63], b) FA suppression of Hpa secretion, thus reducing cardiomyocyte to EC transfer of LPL [41], c) FA detachment of vascular LPL for hepatic degradation [64], and d) FA inactivation of LPL, either directly [65] or through induction of ANGPTL4 [66–68]. With severe diabetes, animals developed hyperlipidemia that was associated with a reduction in heparin-releasable LPL activity in the heart [32]. This occurred in the absence of any change in LPL gene expression [69] suggesting that following diabetes, cardiac LPL activity is mainly modulated by post-translational mechanisms. In this regard, when RNA-seq was performed in diabetic hearts, of the more than fifteen hundred differentially expressed genes, the one that showed the greatest fold change (∼25-fold increase) was ANGPTL4 [30]. Altogether, these results imply that circulating FA has the ability to supress vascular LPL by a host of mechanisms to prevent lipid overload of the heart.
Insulin
Changes in circulating insulin can affect LPL and this response varies with the tissues being studied [70]. Thus, a reduction in insulin after fasting decreases adipocyte LPL but enhances its activity in the heart [71], changes that occurred in the absence of LPL gene or total protein expression [29]. Consequently, the FA that are produced from lipoprotein-TG lipolysis by LPL are directed away from storage in the adipose tissue so that they can fulfill the metabolic demands of cardiomyocytes. As newly synthesized LPL can transfer from myocytes to the vascular EC within 30 min, an augmented vectorial movement of LPL could explain the rapid increase of coronary LPL following fasting [72]. Mechanistically, a reduction in insulin after fasting or STZ-induced diabetes decreases glucose uptake in the heart resulting in activation of AMPK [1, 73, 74] with stimulation of LPL translocation [2] (see detailed review [19]).
Apolipoproteins
Activators of LPL include Apolipoproteins (Apo) C-II and Apo A-V, whereas inhibitors include Apo C-III. Apo-CII is produced primarily in the liver and then incorporated into lipoproteins. On binding to LPL, it promotes conformational changes in the enzyme, allowing the catalytic site of LPL to interact with lipoproteins permitting their hydrolysis [75]. Apo A-V increases the activity of Apo C-II [76, 77].
Oscillations in cardiac LPL following diabetes and its impact on plasma triglycerides
In the clinical setting, plasma LPL activity is determined after infusion of heparin to release HSPG-bound LPL [12, 78]. The downside with this method is that the measured LPL represents enzyme that is released from a host of different tissues (heart, skeletal muscle, adipose tissue). Regarding tissue-specific detection of LPL following diabetes, adipose tissue and skeletal muscle show low levels of enzyme in homogenates [79], with virtually no information available on the cardiac content of this enzyme. Even if heart homogenates are used to determine LPL levels, this would only provide an estimation of total cardiac LPL and would not correctly reflect the enzymatically active LPL at the vascular lumen. Hence, studies in animals have provided the key source of information regarding LPL biology in the diabetic heart. Thus, acute insulin resistance following administration of dexamethasone [80, 81] or hyperglycemia and hypoinsulinemia in rats injected with 55 mg/kg STZ (D55) causes a significant increase of heparin-releasable LPL at the coronary lumen [10, 32, 82, 83]. This increase occurred due to a rapid filling of the unoccupied HSPG-binding sites [70, 82, 84] and was independent of changes in LPL gene and protein expression [82, 85]. Occupation of these empty HSPG-binding sites at the EC surface was mediated by enhanced translocation of LPL [29, 38, 41, 63, 69, 70, 86–91].
For a considerable period, it has been undecided whether the hypertriglyceridemia following diabetes was an outcome of augmented synthesis of VLDL-TG from the liver or a product of reduced clearance of lipoprotein-TG by LPL. In the moderately diabetic D55 animals with 2-fold increase in cardiac LPL activity, circulating TG and NEFA were maintained at levels that were comparable to control animals [30]. Willecke et al. using STZ-diabetic mice revealed that VLDL secretion remained unchanged in these mice [92]. Interestingly however, TG clearance was significantly reduced and was related to a reduction in skeletal muscle, cardiac, and brown adipose tissue LPL mRNA/activity, suggesting that LPL clearance of TG is the more important contributor.
In diabetes, an increase in circulating and intracellular TG are risk factors associated with atherosclerotic cardiovascular disease and cardiac lipotoxicity [93]. It is possible that in D55 heart, LPL derived FA are directed towards oxidative metabolism rather than storage (Figure 2) [30]. Intriguingly, similar to our observations in the moderately diabetic D55 heart, a modest overexpression of LPL in adipose tissue was associated with better glucose and insulin tolerance [94]. When these animals were provided a high fat diet, weight gain was not observed. In fact, dietary lipids did not accumulate in adipose tissue, and the animals displayed amplified energy expenditure. The authors proposed that a moderate increase in adipose LPL has favourable effects on total body energy metabolism. In contrast, we also observed a decline in LPL, both in animals infused with Intralipid [95] and with severe diabetes induced by injecting 100 mg/kg STZ (D100) [10, 30]. As the D100 diabetic animals exhibit elevated plasma FA, we concluded that LPL-mediated FA delivery would be redundant in these circumstances and is “turned off.” Additionally, this reduced cardiac LPL likely contributed to the robust increase in circulating TG. We have previously shown that when FA uptake by LPL action is augmented, this competes with NEFA uptake [23]. Thus, following severe diabetes, when cardiac LPL action is reduced, NEFA uptake and oxidation takes precedence over provision of FAs to the heart from circulating lipoproteins. This excessive supply of NEFA overwhelmed the mitochondrial capacity, leading to a mismatch between FA delivery and utilization, lipid metabolite build-up and cell death (Figure 2) [30]. Thus, approaches that maintain cardiac LPL would be a useful therapeutic approach to preventing cardiac pathology seen following diabetes that is poorly controlled.
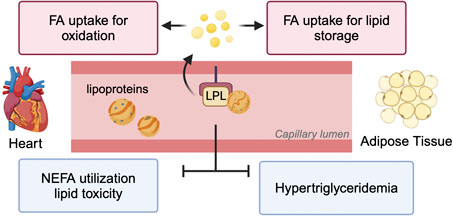
Figure 2. Impact of LPL-derived FA on heart and adipose tissue. LPL positioned at the vascular lumen is responsible for lipoprotein-TG break down to release FA for multiple purposes. These include uptake into the heart for generation of energy and adipose tissue for lipid storage. In pathological conditions like diabetes when LPL activity is reduced in both these tissues, the reduction in TG clearance results in hypertriglyceridemia. Additionally, the heart switches to using NEFA for oxidation leading to excessive TG accumulation and lipotoxicity.
Role of LPL in development of atherosclerosis
Atherosclerosis is defined as a thickening (and loss of elasticity) of the arterial intima as a consequence of lipid accumulation [96]. During this condition, there is narrowing or obstruction of the vessel lumen and thinning of the vessel wall. In preclinical animal models, atherosclerosis is a progressive disease beginning with the development of fatty streaks and potentially leading to complicated atherosclerotic plaques that can rupture, set up thrombosis and occlude the lumen. The clinical manifestations of atherosclerosis are dependent on the site of lesion. Hence, its presence at the coronary arteries leads to angina pectoris and myocardial infarction, at the central nervous system it causes transient cerebral ischemia and stroke whereas its occurrence in the peripheral circulation elicits peripheral vascular disease [96]. The level of plasma cholesterol, and in particular LDL-associated cholesterol, is one of the main risk factors of atherosclerosis [97]. Following LDL infiltration and trapping in the arterial intima with potential oxidative modification, ox-LDL causes endothelial cells to express monocyte chemoattractant protein (MCP-1). MCP-1 attracts monocytes from the vessel lumen into the subendothelial space, one of the very early stages in the development of atherosclerosis. Modified LDL also promotes differentiation of monocytes into macrophages which avidly take up the ox-LDL. This accumulation transforms macrophages into lipid-rich foam cells, that are the hallmark of atherosclerosis [96]. Engorgement of foam cells with lipids causes release of cytokines, and eventually cell death. Macrophage/foam cell-released proteolytic enzymes (matrix metalloproteinases, MMPs) allows for smooth muscle cells from the adjacent media to migrate into the intima, proliferate and secrete fibrous connective tissue (i.e., collagen) and extracellular matrix (smooth muscle cells change their phenotype from contractile to synthetic cells). This makes the lesion harder and contributes to the formation of a fibrous cap (which includes a mixture of macrophages, lipid and cell debris which form a necrotic core). The expanding intima pushes against the endothelial wall of the intima and the fibrous cap is very susceptible to rupture. MMPs also cause a thinning of the fibrous cap with eventual cap destruction along with a host of other events like platelet aggregation and adhesion, thrombosis and clot formation. The rupture of such lesions is believed to be responsible for most cases of unstable angina and acute myocardial infarction. Dislodging of the clot blocks the artery near the plaque or in a more distal and narrower segment causing total or near total occlusion [96, 98].
LPL in macrophages
Given the contribution of LPL in supplying FAs to various tissues for storage and energy generation, in addition to its role in plasma lipoprotein clearance, the action of LPL is considered beneficial. Thus, the contribution of LPL towards the etiology of atherosclerosis is contentious. On the one hand, overexpression of LPL has been shown to protect against diet-induced atherosclerosis in Ldlr−/− and Apoe−/− mice, established animal models to study atherosclerosis [99, 100]. This protective effect of LPL was linked to beneficial changes in plasma lipoproteins. On the other hand, this was not the case when LPL levels were manipulated in macrophages. Specific deletion of macrophage LPL (with no changes in total plasma LPL activity) significantly reduced atherosclerotic lesions in Apoe−/− mice, supporting an important role for macrophage LPL in atherosclerosis [101]. Similarly, overexpression of human LPL in rabbit macrophages accelerated atherosclerotic plaque development, and this occurred in the absence of any changes in plasma lipids [102]. These studies reinforced a pro-atherogenic role for macrophage LPL. It is worth noting that although LPL has abundant expression in highly oxidative tissues, it is also detected in tissues like the kidneys, brain and macrophages where its binding properties are likely more important than its lipolytic activity [103]. Thus, the effect of macrophage LPL on atherosclerosis could be the result of its lipolytic activity and/or its ability to act as a receptor to assist remnant particle uptake. Related to the latter function of LPL, subsequent to lipolysis of lipoproteins, the remnant particles that detach from the endothelial GPIHBP1 platform have inactive LPL bound to them. It has been proposed that this inactive LPL may act as a hepatic receptor ligand to promote lipoprotein uptake [104–106]. When this process occurs in macrophages, LPL acted as a bridging molecule for remnant uptake to increase foam cell formation and lesion progression (Figure 1) [107].
LPL in smooth muscle cells (SMC)
The above discussion brings forward the contribution of macrophages towards foam cell formation and development of atherosclerosis. However, more recent studies have also implicated SMCs in foam cell formation, at least in humans [108]. Thus, in mouse models of atherosclerosis, the lipid milieu along with the resident inflammation recruits’ monocytes across EC to initiate atherosclerosis [109]. In contrast, in human atherosclerosis, SMCs migration from the arterial media occurs before lipid accumulation and in fact SMC make up almost 50% of the foam cells in the plaque lesion [108]. It is possible that SMCs take up lipoprotein remnants through expression of scavenger receptors. However, LPL expression in SMC may also contribute to this mechanism. In vitro, SMC and macrophages synthesize LPL [110] which could act as a co-receptor to facilitate the binding of native and ox-LDL to HSPG (Figure 1) [111]. Interestingly, LPL has been detected in the fibrous cap of the atherosclerotic lesions [112]. Whether SMCs in the atherosclerotic lesion synthesize LPL per se or whether it is transferred from the macrophages is currently unknown. It should be noted that at least in the heart, the translocation of LPL has been reported from cells like the cardiomyocyte to endothelial cells [86]. In support, SMC interact with macrophages both directly and indirectly. For example, in the presence of macrophages, SMC increases their phagocytic activity by enhancing LPL and proteoglycans to promote lipoprotein uptake [113].
LPL as a therapeutic target
Given the importance of LPL in FA delivery to multiple tissues in addition to its contribution to atherosclerotic plaque development, there are a number of pharmaceutical approaches that have been attempted to lower cardiovascular risk by targeting LPL. These include:
Incretins
Oral glucose causes the release of gut hormones like glucagon-like peptide-1 (GLP-1) and glucose-dependent insulinotropic polypeptide (GIP) that amplify glucose-induced insulin secretion in addition to acting via multiple mechanisms to influence blood glucose [114]. Drugs that mimic GLP-1 (e.g., Semaglutide) and/or GIP (e.g., Tirzepatide) are gaining in popularity not only due to their control of blood glucose but also due to their ability to promote weight loss [115, 116]. Intriguingly, cardiovascular outcome trials demonstrate that long-term use of GLP-1 receptor agonists reduce cardiovascular complications of diabetes [117] or obesity [118] by mechanisms that not completely understood. It has been proposed that incretins could offer cardioprotection via their influence on lipid metabolism. In this regard, GLP-1 has been shown to regulate secretion of lipoproteins and cholesterol metabolism [119, 120]. GIP, on the other hand, demonstrated a regulatory role in lipid metabolism that occurred partially via LPL activation. Thus, in cultured 3T3-L1 cells and human adipocytes, GIP stimulated LPL activity in a dose-dependent manner [121–123]. These results were supported by in vivo studies which reported that GIP accelerated chylomicron TG clearance in dogs [124]. Similarly, human studies revealed that GIP infusion significantly increased LPL action, where LPL-derived FA largely contributed to an increase in re-esterification rate and TG storage in adipose tissue of lean individuals [125]. However, unlike adipose tissue, the effects of GIP on cardiac LPL level remain unclear. Given the opposing mechanisms of LPL regulation between adipose tissue and the heart, it is possible that GIP lowers cardiac LPL activity. Interestingly, eliminating GIP receptor signaling protected the heart against experimental myocardial infarction, and this was associated with reduced phosphorylation of HSL and increased cardiac TG storage [126]. As intramuscular TG accumulation is predominantly regulated by the action of HSL and LPL [127], the contribution of cardiac LPL to the altered lipid accumulation and the cardioprotective phenotype of Gipr−/− mice following myocardial infarction would be interesting to study.
Apolipoprotein and angiopoietin-like protein inhibitors
Apolipoprotein C3 (APOC3) and angiopoietin-like protein (e.g. ANGPTL3) are known to inhibit LPL activity directly. Thus, a genetic reduction in APOC3 increases LPL activity, reduces plasma TG and causes a decrease in coronary heart disease [128]. Related to targeting APOC3, two antisense oligonucleotides, Olezarsen and Volanesorsen, are currently in Phase 3 clinical trials to determine their therapeutic potential to lower circulating TG [129, 130]. Similar to APOC3, genetic variants in ANGPTL3 impacts plasma TG [131]. As such, Evinacumab (human monoclonal antibody) is currently on the market to inhibit the action of APOC3 to lower TG levels [132].
Omega-3 fatty acids (icosapent ethyl)
Used in statin-treated patients with elevated TG (≥150 mg/dL), who are at high risk of cardiovascular events due to established cardiovascular disease, or diabetes, and at least one other cardiovascular risk factor. Mechanism of action not completely understood and likely multifactorial and includes a decreased production and accelerated clearance of triglycerides [133]. It decreases VLDL synthesis and secretion by a) reducing hepatic lipogenesis (synthesis of FA from acetyl CoA), b) increasing beta-oxidation of FA and c) inhibiting TG-synthesizing enzymes (e.g., DGAT). It increases VLDL clearance by a) augmenting LPL activity directly or b) indirectly by reducing APOC3, an inhibitor of LPL. It’s a unique form of omega-3 fatty acid (eicosapentaenoic acid) that reduces VLDL-TG. Affects multiple atherosclerotic processes including endothelial function, oxidative stress, foam cell formation, inflammatory response (its anti-inflammatory), platelet aggregation and plaque rupture (it causes plaque regression) [134, 135].
Fibric acid derivatives
Also called fibrates (e.g., fenofibrate and bezafibrate). Function primarily as peroxisome proliferator-activated receptors (PPAR) agonists (stimulates the PPARα receptor), thereby increasing the oxidation of fatty acids in liver (decreases VLDL production) and striated muscle (also kidney and heart). Also increases LPL activity (increased catabolism of circulating TGs increases the rate of clearance of TG) by both transcription upregulation of LPL and down regulation of APOC3 (an inhibitor of LPL) [136, 137].
Statins
They are reversible, competitive inhibitors of HMG-CoA reductase. As a result, there is inhibition of intracellular cholesterol synthesis mainly in the liver. Because a precise amount of cholesterol is required in cells, on decrease of intracellular cholesterol, hepatocytes increase the expression of LDL receptors which then promote the extraction of LDL cholesterol from plasma secondarily. Are also known to influence LPL, especially in patients with Type 2 diabetes [138, 139]. These effects of statins occurred in a tissue specific manner, with an increased LPL production observed in skeletal muscle [140] and a decrease in LPL mass reported in macrophages [141].
Discussion
The enzyme LPL is essential for circulating TG clearance, FA delivery for both oxidation and storage, and for prompting lipoprotein uptake by acting as a receptor. Given these multiple functions, changes in LPL would be expected to have diverse consequences. For example, a decrease in adipose tissue LPL would impede lipoprotein clearance resulting in augmented plasma lipids. In the heart, reduction in LPL, as observed with severe diabetes, causes a switch in substrate utilization to predominantly NEFA. This overwhelms the mitochondrial oxidative capacity leading to TG storage and lipid toxicity. However, depletion of macrophage LPL demonstrated beneficial effects against the development of atherosclerosis. Thus, when attempting to modulate LPL levels, one should consider the tissue and cell type in addition to the disease entity. In this regard, tissue or cell-specific manipulation of LPL offers promise to overcome the cardiac complications associated with obesity and diabetes.
Author contributions
All authors listed have made a substantial, direct, and intellectual contribution to the work and approved it for publication.
Funding
The author(s) declare that financial support was received for the research, authorship, and/or publication of this article. This work was supported by an operating grant from the Canadian Institutes of Health Research [CIHR PJT-178134].
Conflict of interest
The authors declare that the research was conducted in the absence of any commercial or financial relationships that could be construed as a potential conflict of interest.
References
1. An, D, and Rodrigues, B. Role of changes in cardiac metabolism in development of diabetic cardiomyopathy. Am J Physiol Heart circulatory Physiol (2006) 291(4):H1489–506. doi:10.1152/ajpheart.00278.2006
2. Kim, MS, Wang, Y, and Rodrigues, B. Lipoprotein lipase mediated fatty acid delivery and its impact in diabetic cardiomyopathy. Biochim Biophys Acta (BBA) - Mol Cell Biol Lipids (2012) 1821(5):800–8. doi:10.1016/j.bbalip.2011.10.001
3. Camps, L, Reina, M, Llobera, M, Vilaro, S, and Olivecrona, T. Lipoprotein lipase: cellular origin and functional distribution. Am J Physiology-Cell Physiol (1990) 258(4 Pt 1):C673–81. doi:10.1152/ajpcell.1990.258.4.c673
4. Blanchette-Mackie, EJ, Masuno, H, Dwyer, NK, Olivecrona, T, and Scow, RO. Lipoprotein lipase in myocytes and capillary endothelium of heart: immunocytochemical study. Am J Physiology-Endocrinology Metab (1989) 256(6 Pt 1):E818–28. doi:10.1152/ajpendo.1989.256.6.e818
5. Braun, JE, and Severson, DL. Regulation of the synthesis, processing and translocation of lipoprotein lipase. Biochem J (1992) 287(Pt 2):337–47. doi:10.1042/bj2870337
6. Auwerx, J, Leroy, P, and Schoonjans, K. Lipoprotein lipase: recent contributions from molecular biology. Crit Rev Clin Lab Sci (1992) 29(3-4):243–68. doi:10.3109/10408369209114602
7. Beigneux, AP, Allan, CM, Sandoval, NP, Cho, GW, Heizer, PJ, Jung, RS, et al. Lipoprotein lipase is active as a monomer. Proc Natl Acad Sci (2019) 116(13):6319–28. doi:10.1073/pnas.1900983116
8. Kumari, A, Kristensen, KK, Ploug, M, and Winther, AL. The importance of lipoprotein lipase regulation in atherosclerosis. Biomedicines (2021) 9(7):782. doi:10.3390/biomedicines9070782
9. Gunn, KH, Roberts, BS, Wang, F, Strauss, JD, Borgnia, MJ, Egelman, EH, et al. The structure of helical lipoprotein lipase reveals an unexpected twist in lipase storage. Proc Natl Acad Sci U S A. (2020) 117(19):10254–64. doi:10.1073/pnas.1916555117
10. Rodrigues, B, Cam, MC, Jian, K, Lim, F, Sambandam, N, and Shepherd, G. Differential effects of streptozotocin-induced diabetes on cardiac lipoprotein lipase activity. Diabetes (1997) 46(8):1346–53. doi:10.2337/diabetes.46.8.1346
11. Enerback, S, and Gimble, JM. Lipoprotein lipase gene expression: physiological regulators at the transcriptional and post-transcriptional level. Biochim Biophys Acta (Bba) - Lipids Lipid Metab (1993) 1169(2):107–25. doi:10.1016/0005-2760(93)90196-g
12. Eckel, RH, Underhill, LH, and Eckel, RH. Lipoprotein lipase. N Engl J Med (1989) 320(16):1060–8. doi:10.1056/nejm198904203201607
13. Allan, CM, Larsson, M, Jung, RS, Ploug, M, Bensadoun, A, Beigneux, AP, et al. Mobility of "HSPG-bound" LPL explains how LPL is able to reach GPIHBP1 on capillaries. J lipid Res (2017) 58(1):216–25. doi:10.1194/jlr.m072520
14. Ioka, RX, Kang, MJ, Kamiyama, S, Kim, DH, Magoori, K, Kamataki, A, et al. Expression cloning and characterization of a novel glycosylphosphatidylinositol-anchored high density lipoprotein-binding protein, GPI-HBP1. J Biol Chem (2003) 278(9):7344–9. doi:10.1074/jbc.m211932200
15. Young, SG, Davies, BS, Voss, CV, Gin, P, Weinstein, MM, Tontonoz, P, et al. GPIHBP1, an endothelial cell transporter for lipoprotein lipase. J lipid Res (2011) 52(11):1869–84. doi:10.1194/jlr.r018689
16. Davies, BS, Beigneux, AP, Barnes, RH, Tu, Y, Gin, P, Weinstein, MM, et al. GPIHBP1 is responsible for the entry of lipoprotein lipase into capillaries. Cell Metab (2010) 12(1):42–52. doi:10.1016/j.cmet.2010.04.016
17. Weinstein, MM, Yin, L, Tu, Y, Wang, X, Wu, X, Castellani, LW, et al. Chylomicronemia elicits atherosclerosis in mice--brief report. Arteriosclerosis, Thromb Vasc Biol (2010) 30(1):20–3. doi:10.1161/atvbaha.109.196329
18. Young, SG, Davies, BS, Fong, LG, Gin, P, Weinstein, MM, Bensadoun, A, et al. GPIHBP1: an endothelial cell molecule important for the lipolytic processing of chylomicrons. Curr Opin Lipidol (2007) 18(4):389–96. doi:10.1097/mol.0b013e3281527914
19. Shang, R, and Rodrigues, B. Lipoprotein lipase and its delivery of fatty acids to the heart. Biomolecules (2021) 11(7):1016. doi:10.3390/biom11071016
20. Goldberg, IJ, Eckel, RH, and McPherson, R. Triglycerides and heart disease: still a hypothesis? Arteriosclerosis, Thromb Vasc Biol (2011) 31(8):1716–25. doi:10.1161/atvbaha.111.226100
21. Bharadwaj, KG, Hiyama, Y, Hu, Y, Huggins, LA, Ramakrishnan, R, Abumrad, NA, et al. Chylomicron- and VLDL-derived lipids enter the heart through different pathways: in vivo evidence for receptor- and non-receptor-mediated fatty acid uptake. J Biol Chem (2010) 285(49):37976–86. doi:10.1074/jbc.m110.174458
22. He, C, Weston, TA, Jung, RS, Heizer, P, Larsson, M, Hu, X, et al. NanoSIMS analysis of intravascular lipolysis and lipid movement across capillaries and into cardiomyocytes. Cell Metab (2018) 27(5):1055–66.e3. doi:10.1016/j.cmet.2018.03.017
23. Shang, R, Lee, CS, Wang, H, Dyer, R, Noll, C, Carpentier, A, et al. Reduction in insulin uncovers a novel effect of VEGFB on cardiac substrate utilization. Arteriosclerosis, Thromb Vasc Biol (2024) 44(1):177–91. doi:10.1161/atvbaha.123.319972
24. Trent, CM, Yu, S, Hu, Y, Skoller, N, Huggins, LA, Homma, S, et al. Lipoprotein lipase activity is required for cardiac lipid droplet production. J lipid Res (2014) 55(4):645–58. doi:10.1194/jlr.m043471
25. Kudo, N, Barr, AJ, Barr, RL, Desai, S, and Lopaschuk, GD. High rates of fatty acid oxidation during reperfusion of ischemic hearts are associated with a decrease in malonyl-CoA levels due to an increase in 5'-AMP-activated protein kinase inhibition of acetyl-CoA carboxylase. J Biol Chem (1995) 270(29):17513–20. doi:10.1074/jbc.270.29.17513
26. Lopaschuk, GD, Ussher, JR, Folmes, CD, Jaswal, JS, and Stanley, WC. Myocardial fatty acid metabolism in health and disease. Physiol Rev (2010) 90(1):207–58. doi:10.1152/physrev.00015.2009
27. Luiken, JJ, Coort, SL, Willems, J, Coumans, WA, Bonen, A, van der Vusse, GJ, et al. Contraction-induced fatty acid translocase/CD36 translocation in rat cardiac myocytes is mediated through AMP-activated protein kinase signaling. Diabetes (2003) 52(7):1627–34. doi:10.2337/diabetes.52.7.1627
28. An, D, Kewalramani, G, Qi, D, Pulinilkunnil, T, Ghosh, S, Abrahani, A, et al. β-Agonist stimulation produces changes in cardiac AMPK and coronary lumen LPL only during increased workload. Am J Physiol Endocrinol Metab (2005) 288(6):E1120–7. doi:10.1152/ajpendo.00588.2004
29. An, D, Pulinilkunnil, T, Qi, D, Ghosh, S, Abrahani, A, and Rodrigues, B. The metabolic "switch" AMPK regulates cardiac heparin-releasable lipoprotein lipase. Am J Physiol Endocrinol Metab (2005) 288(1):E246–53. doi:10.1152/ajpendo.00211.2004
30. Puri, K, Lal, N, Shang, R, Ghosh, S, Flibotte, S, Dyer, R, et al. Diabetes mellitus severity and a switch from using lipoprotein lipase to adipose-derived fatty acid results in a cardiac metabolic signature that embraces cell death. J Am Heart Assoc (2019) 8(21):e014022. doi:10.1161/jaha.119.014022
31. Wu, Y, Song, P, Xu, J, Zhang, M, and Zou, MH. Activation of protein phosphatase 2A by palmitate inhibits AMP-activated protein kinase. J Biol Chem (2007) 282(13):9777–88. doi:10.1074/jbc.m608310200
32. Wang, Y, Puthanveetil, P, Wang, F, Kim, MS, Abrahani, A, and Rodrigues, B. Severity of diabetes governs vascular lipoprotein lipase by affecting enzyme dimerization and disassembly. Diabetes (2011) 60(8):2041–50. doi:10.2337/db11-0042
33. Fairbanks, MB, Mildner, AM, Leone, JW, Cavey, GS, Mathews, WR, Drong, RF, et al. Processing of the human heparanase precursor and evidence that the active enzyme is a heterodimer. J Biol Chem (1999) 274(42):29587–90. doi:10.1074/jbc.274.42.29587
34. Gingis-Velitski, S, Zetser, A, Kaplan, V, Ben-Zaken, O, Cohen, E, Levy-Adam, F, et al. Heparanase uptake is mediated by cell membrane heparan sulfate proteoglycans. J Biol Chem (2004) 279(42):44084–92. doi:10.1074/jbc.m402131200
35. Pikas, DS, Li, JP, Vlodavsky, I, and Lindahl, U. Substrate specificity of heparanases from human hepatoma and platelets. J Biol Chem (1998) 273(30):18770–7. doi:10.1074/jbc.273.30.18770
36. Abboud-Jarrous, G, Rangini-Guetta, Z, Aingorn, H, Atzmon, R, Elgavish, S, Peretz, T, et al. Site-directed mutagenesis, proteolytic cleavage, and activation of human proheparanase. J Biol Chem (2005) 280(14):13568–75. doi:10.1074/jbc.m413370200
37. Zcharia, E, Metzger, S, Chajek-Shaul, T, Aingorn, H, Elkin, M, Friedmann, Y, et al. Transgenic expression of mammalian heparanase uncovers physiological functions of heparan sulfate in tissue morphogenesis, vascularization, and feeding behavior. FASEB J. (2004) 18(2):252–63. doi:10.1096/fj.03-0572com
38. Wang, Y, Zhang, D, Chiu, AP, Wan, A, Neumaier, K, Vlodavsky, I, et al. Endothelial heparanase regulates heart metabolism by stimulating lipoprotein lipase secretion from cardiomyocytes. Arteriosclerosis, Thromb Vasc Biol (2013) 33(5):894–902. doi:10.1161/atvbaha.113.301309
39. Shafat, I, Ilan, N, Zoabi, S, Vlodavsky, I, and Nakhoul, F. Heparanase levels are elevated in the urine and plasma of type 2 diabetes patients and associate with blood glucose levels. PloS one (2011) 6(2):e17312. doi:10.1371/journal.pone.0017312
40. Katz, A, Van-Dijk, DJ, Aingorn, H, Erman, A, Davies, M, Darmon, D, et al. Involvement of human heparanase in the pathogenesis of diabetic nephropathy. Isr Med Assoc J (2002) 4(11):996–1002.
41. Wang, F, Kim, MS, Puthanveetil, P, Kewalramani, G, Deppe, S, Ghosh, S, et al. Endothelial heparanase secretion after acute hypoinsulinemia is regulated by glucose and fatty acid. Am J Physiol Heart circulatory Physiol (2009) 296(4):H1108–16. doi:10.1152/ajpheart.01312.2008
42. Lee, CS, Zhai, Y, Shang, R, Wong, T, Mattison, AJ, Cen, HH, et al. Flow-induced secretion of endothelial heparanase regulates cardiac lipoprotein lipase and changes following diabetes. J Am Heart Assoc (2022) 11(23):e027958. doi:10.1161/jaha.122.027958
43. Fux, L, Ilan, N, Sanderson, RD, and Vlodavsky, I. Heparanase: busy at the cell surface. Trends in Biochemical Sciences (2009) 34(10):511–9. doi:10.1016/j.tibs.2009.06.005
44. Gingis-Velitski, S, Zetser, A, Flugelman, MY, Vlodavsky, I, and Ilan, N. Heparanase induces endothelial cell migration via protein kinase B/Akt activation. J Biol Chem (2004) 279(22):23536–41. doi:10.1074/jbc.m400554200
45. Cui, HX, Shao, CH, Liu, Q, Yu, WJ, Fang, JP, Yu, WS, et al. Heparanase enhances nerve-growth-factor-induced PC12 cell neuritogenesis via the p38 MAPK pathway. Biochem J (2011) 440:273–82. doi:10.1042/bj20110167
46. Riaz, A, Ilan, N, Vlodavsky, I, Li, JP, and Johansson, S. Characterization of heparanase-induced phosphatidylinositol 3-kinase-AKT activation and its integrin dependence. J Biol Chem (2013) 288(17):12366–75. doi:10.1074/jbc.m112.435172
47. Lee, CS, Shang, R, Wang, F, Khayambashi, P, Wang, H, Araujo, G, et al. Heparanase stimulation of physiological cardiac hypertrophy is suppressed following chronic diabetes resulting in cardiac remodeling and dysfunction. Diabetes (2024):db240217. doi:10.2337/db24-0217
48. Duchesne, L, Octeau, V, Bearon, RN, Beckett, A, Prior, IA, Lounis, B, et al. Transport of fibroblast growth factor 2 in the pericellular matrix is controlled by the spatial distribution of its binding sites in heparan sulfate. PLoS Biol. (2012) 10(7):e1001361. doi:10.1371/journal.pbio.1001361
49. Bishop, JR, Passos-Bueno, MR, Fong, L, Stanford, KI, Gonzales, JC, Yeh, E, et al. Deletion of the basement membrane heparan sulfate proteoglycan type XVIII collagen causes hypertriglyceridemia in mice and humans. PLoS One (2010) 5(11):e13919. doi:10.1371/journal.pone.0013919
50. Chiu, AP, Wan, A, Lal, N, Zhang, D, Wang, F, Vlodavsky, I, et al. Cardiomyocyte VEGF regulates endothelial cell GPIHBP1 to relocate lipoprotein lipase to the coronary lumen during diabetes mellitus. Arteriosclerosis, Thromb Vasc Biol (2016) 36(1):145–55. doi:10.1161/atvbaha.115.306774
51. Wu, SA, Kersten, S, and Qi, L. Lipoprotein lipase and its regulators: an unfolding story. Trends in Endocrinology & Metabolism (2021) 32(1):48–61. doi:10.1016/j.tem.2020.11.005
52. Gin, P, Yin, L, Davies, BS, Weinstein, MM, Ryan, RO, Bensadoun, A, et al. The acidic domain of GPIHBP1 is important for the binding of lipoprotein lipase and chylomicrons. J Biol Chem (2008) 283(43):29554–62. doi:10.1074/jbc.m802579200
53. Goulbourne, CN, Gin, P, Tatar, A, Nobumori, C, Hoenger, A, Jiang, H, et al. The GPIHBP1-LPL complex is responsible for the margination of triglyceride-rich lipoproteins in capillaries. Cell Metab (2014) 19(5):849–60. doi:10.1016/j.cmet.2014.01.017
54. Hultin, M, Savonen, R, Chevreuil, O, and Olivecrona, T. Chylomicron metabolism in rats: kinetic modeling indicates that the particles remain at endothelial sites for minutes. J Lipid Res (2013) 54(10):2595–605. doi:10.1194/jlr.m032979
55. Beigneux, AP, Davies, BS, Gin, P, Weinstein, MM, Farber, E, Qiao, X, et al. Glycosylphosphatidylinositol-anchored high-density lipoprotein-binding protein 1 plays a critical role in the lipolytic processing of chylomicrons. Cell Metab (2007) 5(4):279–91. doi:10.1016/j.cmet.2007.02.002
56. Kristensen, KK, Midtgaard, SR, Mysling, S, Kovrov, O, Hansen, LB, Skar-Gislinge, N, et al. A disordered acidic domain in GPIHBP1 harboring a sulfated tyrosine regulates lipoprotein lipase. Proc Natl Acad Sci USA (2018) 115(26):E6020–E6029. doi:10.1073/pnas.1806774115
57. Mysling, S, Kristensen, KK, Larsson, M, Kovrov, O, Bensadouen, A, Jorgensen, TJ, et al. The angiopoietin-like protein ANGPTL4 catalyzes unfolding of the hydrolase domain in lipoprotein lipase and the endothelial membrane protein GPIHBP1 counteracts this unfolding. eLife (2016) 5:e20958. doi:10.7554/elife.20958
58. Fong, LG, Young, SG, Beigneux, AP, Bensadoun, A, Oberer, M, Jiang, H, et al. GPIHBP1 and plasma triglyceride metabolism. Trends in Endocrinology & Metabolism (2016) 27(7):455–69. doi:10.1016/j.tem.2016.04.013
59. Kroupa, O, Vorrsjo, E, Stienstra, R, Mattijssen, F, Nilsson, SK, Sukonina, V, et al. Linking nutritional regulation of Angptl4, Gpihbp1, and Lmf1 to lipoprotein lipase activity in rodent adipose tissue. BMC Physiol (2012) 12:13. doi:10.1186/1472-6793-12-13
60. Pei-Ling Chiu, A, Wang, F, Lal, N, Wang, Y, Zhang, D, Hussein, B, et al. Endothelial cells respond to hyperglycemia by increasing the LPL transporter GPIHBP1. Am J Physiol Endocrinol Metab (2014) 306(11):E1274–83. doi:10.1152/ajpendo.00007.2014
61. Chiu, AP, Bierende, D, Lal, N, Wang, F, Wan, A, Vlodavsky, I, et al. Dual effects of hyperglycemia on endothelial cells and cardiomyocytes to enhance coronary LPL activity. Am J Physiol Heart circulatory Physiol (2018) 314(1):H82–H94. doi:10.1152/ajpheart.00372.2017
62. Zhang, R, and Zhang, K. An updated ANGPTL3-4-8 model as a mechanism of triglyceride partitioning between fat and oxidative tissues. Prog Lipid Res (2022) 85:101140. doi:10.1016/j.plipres.2021.101140
63. Kim, MS, Wang, F, Puthanveetil, P, Kewalramani, G, Innis, S, Marzban, L, et al. Cleavage of protein kinase D after acute hypoinsulinemia prevents excessive lipoprotein lipase-mediated cardiac triglyceride accumulation. Diabetes (2009) 58(11):2464–75. doi:10.2337/db09-0681
64. Peterson, J, Bihain, BE, Bengtsson-Olivecrona, G, Deckelbaum, RJ, Carpentier, YA, and Olivecrona, T. Fatty acid control of lipoprotein lipase: a link between energy metabolism and lipid transport. Proc Natl Acad Sci (1990) 87(3):909–13. doi:10.1073/pnas.87.3.909
65. Bengtsson, G, and Olivecrona, T. Lipoprotein lipase. Mechanism of product inhibition. Eur J Biochem (1980) 106(2):557–62. doi:10.1111/j.1432-1033.1980.tb04603.x
66. Sukonina, V, Lookene, A, Olivecrona, T, and Olivecrona, G. Angiopoietin-like protein 4 converts lipoprotein lipase to inactive monomers and modulates lipase activity in adipose tissue. Proc Natl Acad Sci (2006) 103(46):17450–5. doi:10.1073/pnas.0604026103
67. Yu, X, Burgess, SC, Ge, H, Wong, KK, Nassem, RH, Garry, DJ, et al. Inhibition of cardiac lipoprotein utilization by transgenic overexpression of Angptl4 in the heart. Proc Natl Acad Sci (2005) 102(5):1767–72. doi:10.1073/pnas.0409564102
68. Lafferty, MJ, Bradford, KC, Erie, DA, and Neher, SB. Angiopoietin-like protein 4 inhibition of lipoprotein lipase: evidence for reversible complex formation. J Biol Chem (2013) 288(40):28524–34. doi:10.1074/jbc.m113.497602
69. Kim, MS, Kewalramani, G, Puthanveetil, P, Lee, V, Kumar, U, An, D, et al. Acute diabetes moderates trafficking of cardiac lipoprotein lipase through p38 mitogen-activated protein kinase-dependent actin cytoskeleton organization. Diabetes (2008) 57(1):64–76. doi:10.2337/db07-0832
70. Pulinilkunnil, T, Abrahani, A, Varghese, J, Chan, N, Tang, I, Ghosh, S, et al. Evidence for rapid "metabolic switching" through lipoprotein lipase occupation of endothelial-binding sites. J Mol Cell Cardiol (2003) 35(9):1093–103. doi:10.1016/s0022-2828(03)00205-0
71. Doolittle, MH, Ben-Zeev, O, Elovson, J, Martin, D, and Kirchgessner, TG. The response of lipoprotein lipase to feeding and fasting. Evidence for posttranslational regulation. J Biol Chem (1990) 265(8):4570–7. doi:10.1016/s0021-9258(19)39601-2
72. Liu, G, and Olivecrona, T. Synthesis and transport of lipoprotein lipase in perfused Guinea pig hearts. Am J Physiology-Heart Circulatory Physiol (1992) 263(2 Pt 2):H438–46. doi:10.1152/ajpheart.1992.263.2.h438
73. Hardie, DG. Minireview: the AMP-activated protein kinase cascade: the key sensor of cellular energy status. Endocrinology (2003) 144(12):5179–83. doi:10.1210/en.2003-0982
74. Hardie, DG, and Carling, D. The AMP-activated protein kinase--fuel gauge of the mammalian cell? Eur J Biochem (1997) 246(2):259–73. doi:10.1111/j.1432-1033.1997.00259.x
75. Wolska, A, Dunbar, RL, Freeman, LA, Ueda, M, Amar, MJ, Sviridov, DO, et al. Apolipoprotein C-II: new findings related to genetics, biochemistry, and role in triglyceride metabolism. Atherosclerosis (2017) 267:49–60. doi:10.1016/j.atherosclerosis.2017.10.025
76. Sharma, V, Ryan, RO, and Forte, TM. Apolipoprotein A-V dependent modulation of plasma triacylglycerol: a puzzlement. Biochim Biophys Acta (BBA) - Mol Cell Biol Lipids (2012) 1821(5):795–9. doi:10.1016/j.bbalip.2011.12.002
77. Merkel, M, Loeffler, B, Kluger, M, Fabig, N, Geppert, G, Pennacchio, LA, et al. Apolipoprotein AV accelerates plasma hydrolysis of triglyceride-rich lipoproteins by interaction with proteoglycan-bound lipoprotein lipase. J Biol Chem (2005) 280(22):21553–60. doi:10.1074/jbc.m411412200
78. Kashiwazaki, K, Hirano, T, Yoshino, G, Kurokawa, M, Tajima, H, and Adachi, M. Decreased release of lipoprotein lipase is associated with vascular endothelial damage in NIDDM patients with microalbuminuria. Diabetes care (1998) 21(11):2016–20. doi:10.2337/diacare.21.11.2016
79. Taskinen, MR, and Nikkila, EA. Lipoprotein lipase activity of adipose tissue and skeletal muscle in insulin-deficient human diabetes. Relation to high-density and very-low-density lipoproteins and response to treatment. Diabetologia (1979) 17(6):351–6. doi:10.1007/bf01236268
80. Qi, D, Pulinilkunnil, T, An, D, Ghosh, S, Abrahani, A, Pospisilik, JA, et al. Single-dose dexamethasone induces whole-body insulin resistance and alters both cardiac fatty acid and carbohydrate metabolism. Diabetes (2004) 53(7):1790–7. doi:10.2337/diabetes.53.7.1790
81. Kewalramani, G, Puthanveetil, P, Kim, MS, Wang, F, Lee, V, Hau, N, et al. Acute dexamethasone-induced increase in cardiac lipoprotein lipase requires activation of both Akt and stress kinases. Am J Physiol Endocrinol Metab (2008) 295(1):E137–47. doi:10.1152/ajpendo.00004.2008
82. Sambandam, N, Abrahani, MA, St Pierre, E, Al-Atar, O, Cam, MC, and Rodrigues, B. Localization of lipoprotein lipase in the diabetic heart: regulation by acute changes in insulin. Arteriosclerosis, Thromb Vasc Biol (1999) 19(6):1526–34. doi:10.1161/01.atv.19.6.1526
83. Sambandam, N, Abrahani, MA, Craig, S, Al-Atar, O, Jeon, E, and Rodrigues, B. Metabolism of VLDL is increased in streptozotocin-induced diabetic rat hearts. Am J Physiol Heart circulatory Physiol (2000) 278(6):H1874–82. doi:10.1152/ajpheart.2000.278.6.h1874
84. Pulinilkunnil, T, Qi, D, Ghosh, S, Cheung, C, Yip, P, Varghese, J, et al. Circulating triglyceride lipolysis facilitates lipoprotein lipase translocation from cardiomyocyte to myocardial endothelial lining. Cardiovasc Res (2003) 59(3):788–97. doi:10.1016/s0008-6363(03)00469-3
85. Pulinilkunnil, T, An, D, Yip, P, Chan, N, Qi, D, Ghosh, S, et al. Palmitoyl lysophosphatidylcholine mediated mobilization of LPL to the coronary luminal surface requires PKC activation. J Mol Cell Cardiol (2004) 37(5):931–8. doi:10.1016/j.yjmcc.2004.07.003
86. Wan, A, and Rodrigues, B. Endothelial cell-cardiomyocyte crosstalk in diabetic cardiomyopathy. Cardiovasc Res (2016) 111(3):172–83. doi:10.1093/cvr/cvw159
87. Kewalramani, G, An, D, Kim, MS, Ghosh, S, Qi, D, Abrahani, A, et al. AMPK control of myocardial fatty acid metabolism fluctuates with the intensity of insulin-deficient diabetes. J Mol Cell Cardiol (2007) 42(2):333–42. doi:10.1016/j.yjmcc.2006.11.010
88. Kim, MS, Wang, F, Puthanveetil, P, Kewalramani, G, Hosseini-Beheshti, E, Ng, N, et al. Protein kinase D is a key regulator of cardiomyocyte lipoprotein lipase secretion after diabetes. Circ Res (2008) 103(3):252–60. doi:10.1161/circresaha.108.178681
89. Pulinilkunnil, T, An, D, Ghosh, S, Qi, D, Kewalramani, G, Yuen, G, et al. Lysophosphatidic acid-mediated augmentation of cardiomyocyte lipoprotein lipase involves actin cytoskeleton reorganization. Am J Physiol Heart circulatory Physiol (2005) 288(6):H2802–10. doi:10.1152/ajpheart.01162.2004
90. Wang, F, Wang, Y, Kim, MS, Puthanveetil, P, Ghosh, S, Luciani, DS, et al. Glucose-induced endothelial heparanase secretion requires cortical and stress actin reorganization. Cardiovasc Res (2010) 87(1):127–36. doi:10.1093/cvr/cvq051
91. Wang, Y, Chiu, AP, Neumaier, K, Wang, F, Zhang, D, Hussein, B, et al. Endothelial cell heparanase taken up by cardiomyocytes regulates lipoprotein lipase transfer to the coronary lumen after diabetes. Diabetes (2014) 63(8):2643–55. doi:10.2337/db13-1842
92. Willecke, F, Scerbo, D, Nagareddy, P, Obunike, JC, Barrett, TJ, Abdillahi, ML, et al. Lipolysis, and not hepatic lipogenesis, is the primary modulator of triglyceride levels in streptozotocin-induced diabetic mice. Arteriosclerosis, Thromb Vasc Biol (2015) 35(1):102–10. doi:10.1161/atvbaha.114.304615
93. Peng, X, and Wu, H. Inflammatory links between hypertriglyceridemia and atherogenesis. Curr Atheroscler Rep (2022) 24(5):297–306. doi:10.1007/s11883-022-01006-w
94. Walton, RG, Zhu, B, Unal, R, Spencer, M, Sunkara, M, Morris, AJ, et al. Increasing adipocyte lipoprotein lipase improves glucose metabolism in high fat diet-induced obesity. J Biol Chem (2015) 290(18):11547–56. doi:10.1074/jbc.m114.628487
95. Qi, D, Kuo, KH, Abrahani, A, An, D, Qi, Y, Heung, J, et al. Acute intralipid infusion reduces cardiac luminal lipoprotein lipase but recruits additional enzyme from cardiomyocytes. Cardiovasc Res (2006) 72(1):124–33. doi:10.1016/j.cardiores.2006.07.022
96. Bjorkegren, JLM, and Lusis, AJ. Atherosclerosis: recent developments. Cell (2022) 185(10):1630–45. doi:10.1016/j.cell.2022.04.004
97. Mortensen, MB, Dzaye, O, Botker, HE, Jensen, JM, Maeng, M, Bentzon, JF, et al. Low-density lipoprotein cholesterol is predominantly associated with atherosclerotic cardiovascular disease events in patients with evidence of coronary atherosclerosis: the western Denmark heart registry. Circulation (2023) 147(14):1053–63. doi:10.1161/circulationaha.122.061010
98. Libby, P, Buring, JE, Badimon, L, Hansson, GK, Deanfield, J, Bittencourt, MS, et al. Atherosclerosis. Nat Rev Dis Primers (2019) 5(1):56. doi:10.1038/s41572-019-0106-z
99. Shimada, M, Ishibashi, S, Inaba, T, Yagyu, H, Harada, K, Osuga, JI, et al. Suppression of diet-induced atherosclerosis in low density lipoprotein receptor knockout mice overexpressing lipoprotein lipase. Proc Natl Acad Sci (1996) 93(14):7242–6. doi:10.1073/pnas.93.14.7242
100. Yagyu, H, Ishibashi, S, Chen, Z, Osuga, J, Okazaki, M, Perrey, S, et al. Overexpressed lipoprotein lipase protects against atherosclerosis in apolipoprotein E knockout mice. J lipid Res (1999) 40(9):1677–85. doi:10.1016/s0022-2275(20)33414-3
101. Takahashi, M, Yagyu, H, Tazoe, F, Nagashima, S, Ohshiro, T, Okada, K, et al. Macrophage lipoprotein lipase modulates the development of atherosclerosis but not adiposity. J lipid Res (2013) 54(4):1124–34. doi:10.1194/jlr.m035568
102. Ichikawa, T, Liang, J, Kitajima, S, Koike, T, Wang, X, Sun, H, et al. Macrophage-derived lipoprotein lipase increases aortic atherosclerosis in cholesterol-fed Tg rabbits. Atherosclerosis (2005) 179(1):87–95. doi:10.1016/j.atherosclerosis.2004.10.044
103. Kirchgessner, TG, LeBoeuf, RC, Langner, CA, Zollman, S, Chang, CH, Taylor, BA, et al. Genetic and developmental regulation of the lipoprotein lipase gene: loci both distal and proximal to the lipoprotein lipase structural gene control enzyme expression. J Biol Chem (1989) 264(3):1473–82. doi:10.1016/s0021-9258(18)94212-2
104. Felts, JM, Itakura, H, and Crane, RT. The mechanism of assimilation of constituents of chylomicrons, very low density lipoproteins and remnants - a new theory. Biochem biophysical Res Commun (1975) 66(4):1467–75. doi:10.1016/0006-291x(75)90524-0
105. Merkel, M, Heeren, J, Dudeck, W, Rinninger, F, Radner, H, Breslow, JL, et al. Inactive lipoprotein lipase (LPL) alone increases selective cholesterol ester uptake in vivo, whereas in the presence of active LPL it also increases triglyceride hydrolysis and whole particle lipoprotein uptake. J Biol Chem (2002) 277(9):7405–11. doi:10.1074/jbc.m107914200
106. Beisiegel, U, Weber, W, and Bengtsson-Olivecrona, G. Lipoprotein lipase enhances the binding of chylomicrons to low density lipoprotein receptor-related protein. Proc Natl Acad Sci (1991) 88(19):8342–6. doi:10.1073/pnas.88.19.8342
107. Gustafsson, M, Levin, M, Skalen, K, Perman, J, Friden, V, Jirholt, P, et al. Retention of low-density lipoprotein in atherosclerotic lesions of the mouse: evidence for a role of lipoprotein lipase. Circ Res (2007) 101(8):777–83. doi:10.1161/circresaha.107.149666
108. Allahverdian, S, Chehroudi, AC, McManus, BM, Abraham, T, and Francis, GA. Contribution of intimal smooth muscle cells to cholesterol accumulation and macrophage-like cells in human atherosclerosis. Circulation (2014) 129(15):1551–9. doi:10.1161/circulationaha.113.005015
109. Swirski, FK, Libby, P, Aikawa, E, Alcaide, P, Luscinskas, FW, Weissleder, R, et al. Ly-6Chi monocytes dominate hypercholesterolemia-associated monocytosis and give rise to macrophages in atheromata. J Clin Invest (2007) 117(1):195–205. doi:10.1172/jci29950
110. Yla-Herttuala, S, Lipton, BA, Rosenfeld, ME, Goldberg, IJ, Steinberg, D, and Witztum, JL. Macrophages and smooth muscle cells express lipoprotein lipase in human and rabbit atherosclerotic lesions. Proc Natl Acad Sci (1991) 88(22):10143–7. doi:10.1073/pnas.88.22.10143
111. Olin, KL, Potter-Perigo, S, Barrett, PH, Wight, TN, and Chait, A. Lipoprotein lipase enhances the binding of native and oxidized low density lipoproteins to versican and biglycan synthesized by cultured arterial smooth muscle cells. J Biol Chem (1999) 274(49):34629–36. doi:10.1074/jbc.274.49.34629
112. Jonasson, L, Bondjers, G, and Hansson, GK. Lipoprotein lipase in atherosclerosis: its presence in smooth muscle cells and absence from macrophages. J lipid Res (1987) 28(4):437–45. doi:10.1016/s0022-2275(20)38694-6
113. Stein, O, Ben-Naim, M, Dabach, Y, Hollander, G, and Stein, Y. Murine macrophages secrete factors that enhance uptake of non-lipoprotein [3H]cholesteryl ester by aortic smooth muscle cells. Biochim Biophys Acta (BBA) - Lipids Lipid Metab (1994) 1212(3):305–10. doi:10.1016/0005-2760(94)90204-6
114. Baggio, LL, and Drucker, DJ. Biology of incretins: GLP-1 and GIP. Gastroenterology (2007) 132(6):2131–57. doi:10.1053/j.gastro.2007.03.054
115. Wilding, JPH, Batterham, RL, Calanna, S, Davies, M, Van Gaal, LF, Lingvay, I, et al. Once-weekly semaglutide in adults with overweight or obesity. N Engl J Med (2021) 384(11):989–1002. doi:10.1056/nejmoa2032183
116. Jastreboff, AM, Aronne, LJ, Ahmad, NN, Wharton, S, Connery, L, Alves, B, et al. Tirzepatide once weekly for the treatment of obesity. N Engl J Med (2022) 387(3):205–16. doi:10.1056/nejmoa2206038
117. Marso, SP, Bain, SC, Consoli, A, Eliaschewitz, FG, Jodar, E, Leiter, LA, et al. Semaglutide and cardiovascular outcomes in patients with type 2 diabetes. N Engl J Med (2016) 375(19):1834–44. doi:10.1056/nejmoa1607141
118. Lincoff, AM, Brown-Frandsen, K, Colhoun, HM, Deanfield, J, Emerson, SS, Esbjerg, S, et al. Semaglutide and cardiovascular outcomes in obesity without diabetes. N Engl J Med (2023) 389(24):2221–32. doi:10.1056/nejmoa2307563
119. Farr, S, Baker, C, Naples, M, Taher, J, Iqbal, J, Hussain, M, et al. Central nervous system regulation of intestinal lipoprotein metabolism by glucagon-like peptide-1 via a brain-gut Axis. Arteriosclerosis, Thromb Vasc Biol (2015) 35(5):1092–100. doi:10.1161/atvbaha.114.304873
120. Wu, YR, Shi, XY, Ma, CY, Zhang, Y, Xu, RX, and Li, JJ. Liraglutide improves lipid metabolism by enhancing cholesterol efflux associated with ABCA1 and ERK1/2 pathway. Cardiovasc diabetology (2019) 18(1):146. doi:10.1186/s12933-019-0954-6
121. Eckel, RH, Fujimoto, WY, and Brunzell, JD. Gastric inhibitory polypeptide enhanced lipoprotein lipase activity in cultured preadipocytes. Diabetes (1979) 28(12):1141–2. doi:10.2337/diabetes.28.12.1141
122. Kim, SJ, Nian, C, and McIntosh, CH. Activation of lipoprotein lipase by glucose-dependent insulinotropic polypeptide in adipocytes. J Biol Chem (2007) 282(12):8557–67. doi:10.1074/jbc.m609088200
123. Kim, SJ, Nian, C, and McIntosh, CH. GIP increases human adipocyte LPL expression through CREB and TORC2-mediated trans-activation of the LPL gene. J lipid Res (2010) 51(11):3145–57. doi:10.1194/jlr.m006841
124. Wasada, T, McCorkle, K, Harris, V, Kawai, K, Howard, B, and Unger, RH. Effect of gastric inhibitory polypeptide on plasma levels of chylomicron triglycerides in dogs. J Clin Invest (1981) 68(4):1106–7. doi:10.1172/jci110335
125. Asmar, M, Asmar, A, Simonsen, L, Gasbjerg, LS, Sparre-Ulrich, AH, Rosenkilde, MM, et al. The gluco- and liporegulatory and vasodilatory effects of glucose-dependent insulinotropic polypeptide (GIP) are abolished by an antagonist of the human GIP receptor. Diabetes (2017) 66(9):2363–71. doi:10.2337/db17-0480
126. Ussher, JR, Campbell, JE, Mulvihill, EE, Baggio, LL, Bates, HE, McLean, BA, et al. Inactivation of the glucose-dependent insulinotropic polypeptide receptor improves outcomes following experimental myocardial infarction. Cell Metab (2018) 27(2):450–60.e6. doi:10.1016/j.cmet.2017.11.003
127. Oscai, LB, Essig, DA, and Palmer, WK. Lipase regulation of muscle triglyceride hydrolysis. J Appl Physiol (1985)1990) 69(5):1571–7. doi:10.1152/jappl.1990.69.5.1571
128. TG and HDL Working Group of the Exome Sequencing Project, National Heart, Lung, and Blood Institute, Crosby, J, Peloso, GM, Auer, PL, Crosslin, DR, Stitziel, NO, Lange, LA, et al. Loss-of-function mutations in APOC3, triglycerides, and coronary disease. N Engl J Med. (2014) 371(1):22–31. doi:10.1056/nejmoa1307095
129. Witztum, JL, Gaudet, D, Freedman, SD, Alexander, VJ, Digenio, A, Williams, KR, et al. Volanesorsen and triglyceride levels in familial chylomicronemia syndrome. N Engl J Med (2019) 381(6):531–42. doi:10.1056/nejmoa1715944
130. Bergmark, BA, Marston, NA, Prohaska, TA, Alexander, VJ, Zimerman, A, Moura, FA, et al. Olezarsen for hypertriglyceridemia in patients at high cardiovascular risk. N Engl J Med (2024) 390(19):1770–80. doi:10.1056/nejmoa2402309
131. Willer, CJ, Schmidt, EM, Sengupta, S, Peloso, GM, Gustafsson, S, Kanoni, S, et al. Discovery and refinement of loci associated with lipid levels. Nat Genet (2013) 45(11):1274–83. doi:10.1038/ng.2797
132. Raal, FJ, Rosenson, RS, Reeskamp, LF, Hovingh, GK, Kastelein, JJP, Rubba, P, et al. Evinacumab for homozygous familial hypercholesterolemia. N Engl J Med (2020) 383(8):711–20. doi:10.1056/nejmoa2004215
133. Kastelein, JJ, Maki, KC, Susekov, A, Ezhov, M, Nordestgaard, BG, Machielse, BN, et al. Omega-3 free fatty acids for the treatment of severe hypertriglyceridemia: the EpanoVa fOr Lowering Very high triglyceridEs (EVOLVE) trial. J Clin Lipidol (2014) 8(1):94–106. doi:10.1016/j.jacl.2013.10.003
134. Bhatt, DL, Steg, PG, Miller, M, Brinton, EA, Jacobson, TA, Ketchum, SB, et al. Cardiovascular risk reduction with icosapent ethyl for hypertriglyceridemia. N Engl J Med (2019) 380(1):11–22. doi:10.1056/nejmoa1812792
135. Moon, JH, Kim, K, and Choi, SH. Lipoprotein lipase: is it a magic target for the treatment of hypertriglyceridemia. Endocrinol Metab (Seoul) (2022) 37(4):575–86. doi:10.3803/enm.2022.402
136. Schoonjans, K, Peinado-Onsurbe, J, Lefebvre, AM, Heyman, RA, Briggs, M, Deeb, S, et al. PPARalpha and PPARgamma activators direct a distinct tissue-specific transcriptional response via a PPRE in the lipoprotein lipase gene. EMBO J (1996) 15(19):5336–48. doi:10.1002/j.1460-2075.1996.tb00918.x
137. Staels, B, Vu-Dac, N, Kosykh, VA, Saladin, R, Fruchart, JC, Dallongeville, J, et al. Fibrates downregulate apolipoprotein C-III expression independent of induction of peroxisomal acyl coenzyme A oxidase. A potential mechanism for the hypolipidemic action of fibrates. J Clin Invest (1995) 95(2):705–12. doi:10.1172/jci117717
138. Endo, K, Miyashita, Y, Saiki, A, Oyama, T, Koide, N, Ozaki, H, et al. Atorvastatin and pravastatin elevated pre-heparin lipoprotein lipase mass of type 2 diabetes with hypercholesterolemia. J Atheroscler Thromb (2004) 11(6):341–7. doi:10.5551/jat.11.341
139. Isley, WL, Harris, WS, and Miles, JM. The effect of high-dose simvastatin on free fatty acid metabolism in patients with type 2 diabetes mellitus. Metabolism (2006) 55(6):758–62. doi:10.1016/j.metabol.2006.01.013
140. Ohira, M, Endo, K, Saiki, A, Miyashita, Y, Terai, K, Murano, T, et al. Atorvastatin and pitavastatin enhance lipoprotein lipase production in L6 skeletal muscle cells through activation of adenosine monophosphate-activated protein kinase. Metabolism (2012) 61(10):1452–60. doi:10.1016/j.metabol.2012.03.010
Keywords: cardiomyopathy, lipoprotein, atherosclerosis, fatty acid metabolism, cardiomyocytes
Citation: Shang R and Rodrigues B (2024) Lipoprotein lipase as a target for obesity/diabetes related cardiovascular disease. J. Pharm. Pharm. Sci 27:13199. doi: 10.3389/jpps.2024.13199
Received: 28 April 2024; Accepted: 02 July 2024;
Published: 16 July 2024.
Edited by:
John Reyes Ussher, University of Alberta, CanadaCopyright © 2024 Shang and Rodrigues. This is an open-access article distributed under the terms of the Creative Commons Attribution License (CC BY). The use, distribution or reproduction in other forums is permitted, provided the original author(s) and the copyright owner(s) are credited and that the original publication in this journal is cited, in accordance with accepted academic practice. No use, distribution or reproduction is permitted which does not comply with these terms.
*Correspondence: Rui Shang, cnNoYW5nQGx1bmVuZmVsZC5jYQ==; Brian Rodrigues, cm9kcmlndWVAbWFpbC51YmMuY2E=