- 1Área de Edafoloxía e Química Agrícola, Facultade de Ciencias, Universidade de Vigo, Ourense, Galicia, Spain
- 2Departamento de Edafoloxía e Química Agrícola, Escola Politécnica Superior, Enxeñaría Universidade de Santiago de Compostela, Campus University, Lugo, Galicia, Spain
- 3Departamento de Bioquímica del Suelo Misión Biológica de Galicia (MBG-CSIC), Santiago de Compostela, Galicia, Spain
A laboratory work has been carried out to determine the tolerance of soil bacterial communities to Ni and Zn and co-tolerance to tetracycline antibiotics (chlortetracycline (CTC), oxytetracycline (OTC) and tetracycline (TC)) in soils individually spiked with five different concentrations of Ni or Zn (1,000, 750, 500, 250, and 125 mg kg−1), and an uncontaminated (0 mg kg−1) control soil. The PICT parameter (pollution-induced community tolerance) was estimated for the bacterial community using the tritium (3H)-labeled leucine incorporation technique, and the values corresponding to log IC50 were used as toxicity index. The mean log IC50 values observed in the uncontaminated soil samples indicate that Zn (with log IC50 = −2.83) was more toxic than Ni (log IC50 = −2.73). In addition, for the soil with the lowest carbon content (C = 1.9%), Ni-contaminated samples showed increased tolerance when the Ni concentrations added were ≥500 mg kg−1, while for the soils with higher carbon content (between 5.3% and 10.9%) tolerance increased when Ni concentrations added were ≥1,000 mg kg−1. Regarding the soils contaminated with Zn, tolerance increased in all the soils studied when the Zn concentrations added were ≥125 mg kg−1, regardless of the soil carbon content. The co-tolerance increases obtained after exposure of the bacterial suspension to TC, OTC and CTC showed an identical behavior within these tetracycline antibiotics. However, it was dependent on the heavy metal tested (Ni or Zn). In the case of soils 1 (C = 1.1%) and 2 (C = 5.3%), the soil bacterial communities showed increases in co-tolerance to TC, OTC and CTC for Ni concentrations added of ≥125 mg kg−1, while for soil 3 (with C = 10.9%) co-tolerance took place when Ni was added at ≥1,000 mg kg−1. However, in soils contaminated with Zn, increases in co-tolerance to CTC, OTC and TC occurred at Zn concentrations added of ≥125 mg kg−1 for the 3 soils tested. These results can be considered relevant when anticipating possible environmental repercussions related to the simultaneous presence of various types of pollutants, specifically certain heavy metals and antibiotics.
Introduction
Soil microorganisms are key components in ecosystem, by their relevance in process such as organic matter recycling or cycling of nutrients. The correct maintenance of biological activity in soils is considered a key characteristic to guarantee the functions of an ecosystem and its sustainable productivity (Swift, 1994). The presence of contaminants in the soil can endanger the proper functioning of its biological activity since soil microorganisms are more sensitive than other organisms to some contaminants such as heavy metals (Brookes, 1995; Giller et al., 1999). Although, heavy metals are of moderate to high physiological importance as they are essential micronutrients required for various cellular components and functions, such as Zn for DNA and RNA polymerase, and Ni for urease (Seiler and Berendonk, 2012), the presence of these compounds in high concentrations can bring negative effects. Heavy metals can be naturally present in soils, although there are also various anthropic activities, such as agricultural, livestock, and industrial production, as well as mining, which can promote a high entry of these substances into terrestrial ecosystems (Kabata-Pendias, 2000; Abdu et al., 2017). Agricultural soils can suffer from high concentrations of heavy metals due to the repeated application of various amendments, such as sewage sludge, phosphate fertilizers, nitrate fertilizers, manure, lime, and composted residues. As indicated in various publications (European Union, 2006; Wu et al., 2013) heavy metals are frequently used in livestock production, with many commercial feedings being added with Zn, Cu and other essential elements, mainly used with the aim of preventing different diseases.
In addition to the use of heavy metals in livestock production, the use of veterinary antibiotics to treat infectious diseases in animals or as growth promoters has been implemented in the last century (Sapkota et al., 2008). In addition, the use of veterinary antibiotics to treat infectious diseases is common in livestock production. The most widely used antibiotics in Europe are tetracyclines, among which chlortetracycline (CTC), oxytetracycline (OTC), and tetracycline (TC) stand out (European Medicines Agency, 2016). Once administered to cattle, antibiotics and heavy metals are poorly absorbed by the animal intestine and a high percentage of these compounds are excreted and accumulated in feces and urine (Sarmah et al., 2006; Zhang et al., 2012; Hejna et al., 2018). Tetracycline antibiotics and heavy metals tend to accumulate in agricultural soils due to frequent spreading of manures and slurries as organic amendments (Hamscher et al., 2002; He et al., 2009), being a widespread problem throughout the world.
The toxicity of antibiotics and heavy metals on soil microorganisms depends on their concentration and their chemical form (Nies, 1999). In this regard, there are several studies that have evaluated the toxicity of heavy metals in soil microbial communities (Hattori, 1992; Giller et al., 1998; Giller et al., 2009; Abdu et al., 2017). On the other hand, there are also previous studies focused on studying the effects of antibiotics on soil microorganisms (Thiele-Bruhn, 2005; Toth et al., 2011; Caban et al., 2018; Urra et al., 2019), however, there are few studies that focus on the study of tetracyclines in soil bacterial communities, such as Santás-Miguel et al. (2020a); Santás-Miguel et al., 2020c; Santás-Miguel et al., 2020d), or on the temporal evolution of various antibiotics affected by environmental conditions, such as Rodríguez-López et al. (2022). On the other hand, several works evaluated the co-tolerance to antibiotics of soil bacterial communities, at the functional and genetic level, in soils contaminated with heavy metals (Bååth, 1989; Berg et al., 2010; Oyetibo et al., 2010; Ji et al., 2012; Dickinson et al., 2019; Santás-Miguel et al., 2020b; Santás-Miguel et al., 2022). However, few studies focused on the concentration levels present in soils that would be the starting point to cause increases in co-tolerance of soil bacterial communities to antibiotics when simultaneously contaminated with heavy metals (Fernández-Calviño and Bååth, 2013; Song et al., 2017; Santás-Miguel et al., 2020b). Knowledge of these concentrations would shed light on the vulnerability of soils contaminated with heavy metals to the presence of bacteria resistant to antibiotics, without previously having been in contact with them. These antibiotic-resistant bacteria, through horizontal gene transfer, can confer resistance to human pathogens, becoming a threat to public and environmental health (Davies, 1994; Knapp et al., 2010; Serwecińska, 2020).
Therefore, the main objective of this study was to determinate the concentrations from which increases in tolerance to Ni and Zn and co-tolerance to tetracycline antibiotics (CTC, OTC, and TC) occur in 3 agricultural soils contaminated separately with 5 different concentrations of Ni and Zn (1,000, 750, 500, 250, and 125 mg kg−1) compared with a control (0 mg kg−1). This study could shed light on the vulnerability of soils contaminated with heavy metals to achieve increased tolerance to antibiotics. This information could facilitate an ulterior design and implementation of appropriate management practices to mitigate these undesirable effects.
Material and Methods
Chemicals
The metals in this research were Zn (as Zn(NO3)2 6H2O, CAS; 10196–18-6) and Ni (as Ni(NO3)2 6H2O, CAS; 13478-00-7). They were supplied by Panreac (Barcelona, Spain).
The antibiotics used in the tests were chlortetracycline hydrochloride (C22H23ClN2O8) (≥97% in purity; CAS 64-72-2), oxytetracycline hydrochloride (C22H24N2O9) (≥95% in purity; CAS 2058-46-0), and tetracycline hydrochloride (C22H24N2O8) (≥95% in purity; CAS 64-75-5), from Sigma–Aldrich (Steinheim, Germany).
Soil Samples
Three agricultural soil samples from A Limia area in Galicia (NW of Spain), were selected form a set previously characterized (Conde-Cid et al., 2018). These soil samples were previously used by Santás-Miguel et al. (2020c) and Santás-Miguel et al. (2020d) for measuring the bacterial growth in soils polluted with tetracycline antibiotics. The methods used to analyze these soils are included in Supplementary Material S1.
The main characteristics of the soils studied are shown in Supplementary Table S1. Briefly, the 3 soils present similar values of pH measured in water, ranging 4.5–4.8. However, they show very different organic carbon contents, with values between 1.1% and 10.9%, while DOC varies between 210.5 and 572 mg kg−1. The sand, silt and clay contents varied between 40.6 and 70.4, between 11.9 and 25.8, and between 17.7 and 33.6 respectively. Therefore, the texture of soil samples was sandy loam for soil 1, clay loam for soil 2 and sandy clay loam for soil 3. The cation exchange capacity ranged between 4.1 and 11.7 cmolc kg−1. The Alo and Feo values ranged 855–3,535 mg kg−1 and 1,150–2080 mg kg−1, respectively. Total Ni and Zn contents ranged 14.0–19.7 and 64–141 mg kg−1, respectively. These values are similar to those found by Macías and Calvo (2008) in uncontaminated soils in the area. The concentration of bioavailable heavy metals to most heavy metal concentration added (1,000 mg kg−1) at days 0 and 42 is shown in Supplementary Table S2. The concentrations of bioavailable heavy metals at day 0 in soil 1, 2 and 3, determined in polluted soil samples extracted with CaCl2 were 413.6, 365.9 and 356.6 mg kg−1, respectively, for Ni and 558.5, 506.6 and 585.4 mg kg−1 respectively, for Zn. On the other hand, the soil samples extracted with EDTA in soil 1, 2 and 3 at day 0, they were 900.8, 560.6 and 503.1 mg kg−1 for Ni, respectively, and 878.6, 692.2 and 615.2 mg kg−1 for Zn (Supplementary Table S2). The bioavailable concentrations determined in polluted soil samples at day 42 of incubation in soil 1, 2 and 3 were 288.2, 271.5 and 257.7 mg kg−1 for Ni and were 527.8, 490.9 and 505.5 mg kg−1 for Zn, when extracted with CaCl2, while they were 731.2, 367.8 and 333.5 mg kg−1 corresponding to Ni and 788.3, 528.7 and 517.9 mg kg−1 corresponding to Zn, when extracted with EDTA (Supplementary Table S2).
Experimental Design
Each of the 3 soil samples were introduced into polyethylene bottles of 500 mL (84 g per bottle) and moistened up to 70% of their water holding capacity, being incubated for 1 week (at 22°C, in the dark), as indicated by Meisner et al. (2013) to facilitate the correct growth of the soil bacterial communities after adjustment of moisture. After this time, the soil samples were distributed in 6 polypropylene tubes of 50 mL (14 g wet weight) and separately spiked with 5 solution with different concentrations of Ni or Zn to reach a final concentration of 1,000, 750, 500, 250, and 125 mg kg−1, also preparing an uncontaminated control soil with water (0 mg kg−1). Then, for each metal (Ni or Zn), the 6 microcosms were distributed in 18 polypropylene tubes of 50 mL (6 microcosms x 3 replication), with 4.6 g (expressed in dry weight) included in each of the tubes, and then incubated (in dark conditions) during 42 days, being a total of 108 microcosms for 3 soil samples and each heavy metal (Ni or Zn). A schematic description of the experimental design is shown in Supplementary Figure S1. The procedure detailed in Díaz-Raviña et al. (1994) and in Díaz-Raviña and Baath. (1996) was followed to determine the bacterial community tolerance (although with some modifications), complemented by the details published by Díaz-Raviña and Bååth. (2001) and Díaz-Raviña et al. (1994) as regards the leucine incorporation method. For this, soil samples corresponding to each microcosm were added with distilled water (at 1:20 (w/v)), shaking with a multivortex (at maximum intensity) for 3 min. Then, the soil + water mixture was centrifuged during 10 min at 1,000 x g, obtaining the soil bacterial suspension (supernatant). Volumes of 1.50 mL of the supernatant were transferred to Eppendorf vials of 2 mL, in which 150 µL of pollutant (heavy metal or antibiotic) were subsequently added. The different concentrations of heavy metals (Ni and Zn) and antibiotics (CTC, OTC, and TC) added to the bacterial suspension plus a control sample (with only water added) made a total of 8 concentrations of pollutants used for each compound and soil sample. The final heavy metal concentrations added in liquid to bacterial suspension ranged between 10–2 and 10–5 mol L−1, while for the antibiotics ranged between 400 and 9.7 × 10−2 mg L−1. The bacterial community growth was estimated after a pre-incubation step of 24 h of bacterial suspension containing the different antibiotics concentrations added before performing the leucine incorporation assay (Berg et al., 2010; Fernández-Calviño and Bååth, 2013). On each micro-centrifugation tube, [3H] leucine incorporation was then measured as follows: 0.2 µL [3H] Leu (3.7 MBq mL−1 and 0.574 TBq mmol−1; Amersham) incorporated with non-labeled Leu into each of the tubes, giving 275 nM Leu in the bacterial suspensions. To stop bacterial growth 75 µL of trichloroacetic acid (at 100%) were added after 3 h of incubation. The indications from Díaz-Raviña and Bååth. (2001) for washing, whereas a liquid scintillation counter (Tri-Carb 2810 TR, PerkinElmer, USA) was used for the radioactivity measurement. For determining the co-tolerance, the results of bacterial growth that were obtained after the exposure of the bacterial suspension to 8 concentrations (from o to 400 mg L−1) of the tetracycline antibiotics (CTC, OTC, and TC, separately), were normalized with respect to the control, obtaining dose-response curves for the different soil samples contaminated with the various Ni or Zn concentrations added (1,000, 750, 500, 250, and 125 mg kg−1, and the unpolluted control sample).
Data Analyses
To estimate the tolerance of the bacterial community to Ni and Zn, and to CTC, OTC and TC, the logarithm of the concentration that resulted in 50% inhibition of bacterial community growth (log IC50) was used. Log IC50 was calculated using a logistic model, detailed below (Sebaugh, 2011):
Where Y is the level of Leu incorporation (measured), X is the logarithm of the concentration of the substance (Ni and Zn, or CTC, OTC and TC) added to the bacterial suspension, a is the log IC50, c the bacterial growth in absence of the toxics, and b is a slope parameter indicative of the inhibition rate. High log IC50 values indicate higher community tolerance, whereas lower values indicate higher toxicity to the bacterial community.
Results and Discussion
Tolerance of Soil Bacterial Communities to Ni and Zn
The results of bacterial growth measured for each dose of Ni and Zn [1,000, 750, 500, 250, 125, and 0 mg kg−1)] were obtained after exposing the bacterial suspensions to the various concentrations of heavy metals (which expressed in molar concentrations went from 0 to 10–2 mol L−1). After being normalized compared to the control, dose-response curves were obtained for the 3 tested soils (Supplementary Figure S2). These dose-response curves were of sigmoidal type, that is, low concentrations of added heavy metal do not cause inhibition of bacterial growth, however, as the heavy metal concentration added is increased, bacterial growth is progressively inhibited. The dose-response curves show a shift to the right compared to the control, which suggests increases in the tolerance of the bacterial communities to the both heavy metals tested.
The dose-response curves presented good fits (Supplementary Tables S3, S4) to the logistic model (Eq. 1) with R2 from 0.873 to 0.996 (Mean = 0.960) in soils contaminated with Ni, and R2 from 0.824 to 0.999 (Mean = 0.975) for soils contaminated with Zn. From the dose-response curves obtained for Ni and Zn in the 3 soils studied, the log IC50 values were estimated for the 6 concentrations tested, as represented in Figure 1. The log IC50 values obtained for the control samples (0 mg kg−1) for each soil show scores between −3.05 and −2.36 (mean = −2.73) for Ni, and between −2.99 and −2.69 (mean = −2.83) for Zn. Observing the mean log IC50 values, the concentration that inhibits 50% of the bacterial population is slightly lower for Zn than that observed for Ni, indicating that Zn seems has higher toxicity than Ni on bacterial communities in the soil samples studied. The log IC50 values obtained in the present study for the control sample are similar to those found in previous works by Santás-Miguel et al. (2022), with log IC50 values of −2.56 for Ni and −2.89 for Zn.
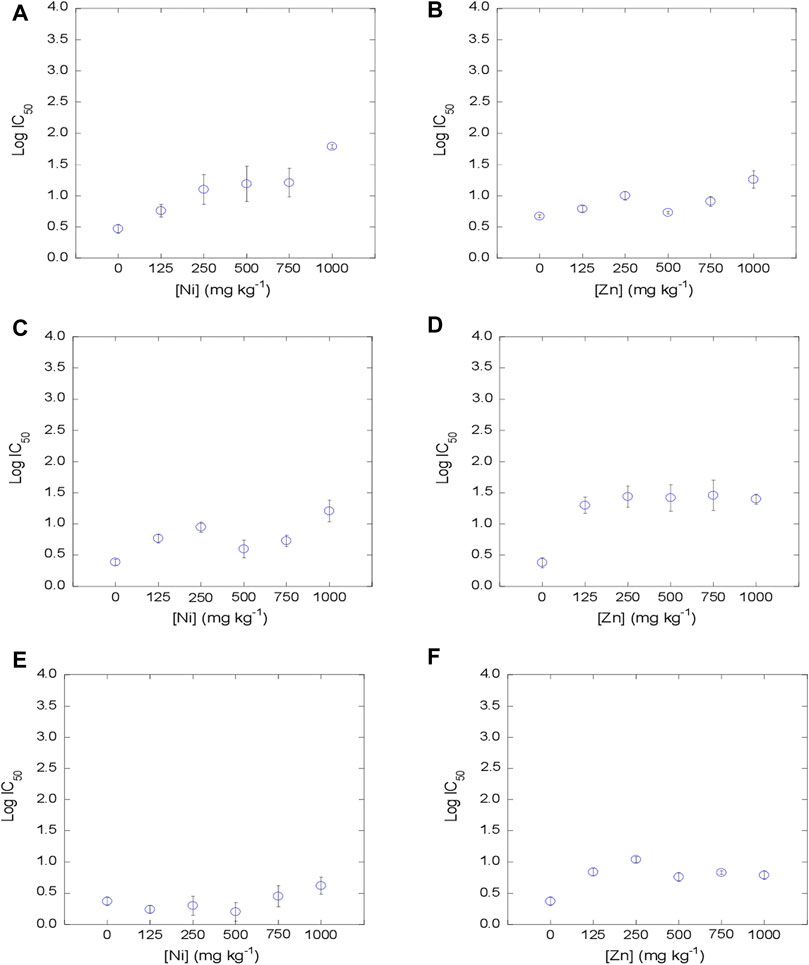
FIGURE 1. Evolution of bacterial community tolerance to Ni and Zn (as log IC50), for increasing Ni and Zn concentrations in the soil (0, 125, 250, 500, 750 and 1,000 mg kg−1). (A, B): soil 1; (C, D): soil 2, (E, F): soil 3.
The increases in tolerance to heavy metals were different depending on the soil and the heavy metal tested. Soil bacterial communities show increased tolerance to Ni at concentrations ≥500 mg kg−1 for soil 1 (C = 1.1%) with respect to the control. However, increases in tolerance to Ni in soils with higher carbon contents (5.3% for soil 2, and 10.9% for soil 3) occur at high metal concentrations added (≥1,000 mg kg−1). On the other hand, regarding Zn, the increase in tolerance with respect to this metal is not dependent on the carbon content of the soil, since there is an increase in tolerance to Zn with respect to the control in all soils at concentrations ≥125 mg kg−1.
The results of the current study are similar to those shown by various authors who also observed increased tolerance to Ni and Zn in soils polluted with different concentrations of these metals (Duxbury and Bicknell, 1983; Díaz-Raviña et al., 1994; Díaz-Raviña and Bååth, 2001; Almås et al., 2004; Davis et al., 2004; Stefanowicz et al., 2009; Zhong et al., 2021; Santás-Miguel et al., 2022). For example, Díaz-Raviña et al. (1994) after 9 months of incubation observed a rise in tolerance with respect to the control for all the concentrations of Zn and Ni tested in agricultural soils from southern Sweden, which were polluted with 1878 mg kg−1 of Ni and between 1,046 and 2093 mg kg−1 of Zn. Stefanowicz et al. (2009) measured the tolerance of bacterial communities in soils near galvanized electricity pylons contaminated with Zn using the Biolog plate technique and observed an increased tolerance to Zn at concentrations ≥300 mg kg−1.
Observing the results obtained in the present research, it is shown that Ni toxicity on soil bacterial communities has a slight dependence on soil carbon content, while it does not happen for Zn. In this regard, it should be bear in mind that the affinity shown by heavy metals for organic matter increases with pH, therefore, at acidic pH, the affinity will be lower than at basic pH (Spark et al., 1997). Taking into account that the pH of the soils here studied is between 4.5 and 4.8, the results obtained may be due to the affinity that these metals have for the organic matter of the soil. In fact, it is classified, according to (Gluskoter, 1977), as a low affinity for Zn and medium affinity for Ni. This difference in heavy metals affinity for soil organic matter has been demonstrated by a large number of authors (Khan and Schnitzer, 1978; McBride, 1994; Rule, 1999; Sauvé et al., 2003; Refaey et al., 2014). In this sense, Piccolo (1989) measured the effect of humic acids on the immobilization of different heavy metals in soils and observed the following sequence: Pb > Cu > Cd > Ni > Zn, with Zn being the metal with the highest solubility followed by Ni and, therefore, having greater bioavailability in soils than other heavy metals. However, considering that other studies such Milne et al. (2003) showed a slightly lower Ni affinity for humic acids than Zn, other causes different than affinities for organic matter cannot be discarded. Thus, log IC50 values found in non-amended soil samples showed a slightly higher toxicity of Zn than Ni on bacterial communities (Supplementary Tables S3, S4).
The high bioavailability of these metals in the soil causes the bacterial communities in the soil to be exposed to this contaminant and therefore the selection pressure exerted on the communities is higher (Blanck, 2002). Zn, therefore, exerts higher selection pressure than Ni, which caused that metal tolerance increases take place from lower Zn concentrations than those needed for Ni.
Co-Tolerance of Soil Bacterial Communities to Tetracycline Antibiotics in Ni and Zn Polluted Soils
The results of bacterial growth obtained after exposure of the bacterial suspension to 8 concentrations of the three tetracycline antibiotics (400–0 mg L−1), added separately, were normalized with respect to the control, giving sigmoidal-type dose-response curves for the different soil samples contaminated with the various concentrations of Ni and Zn (1,000, 750, 500, 250, 125, and 0 mg kg−1) (Supplementary Figures S3–S5). The dose-response curves show a clear shift to the right for most heavy metals concentrations, indicating that there is a clear increase in co-tolerance to CTC, OTC and TC in most of the soils contaminated with the different Ni and Zn concentrations.
The dose-response curves were satisfactorily fitted to the logistic model, with R2 values between 0.890 and 0.999 (mean = 0.983) for the 3 tetracyclines in Ni-contaminated soils, and with R2 values between 0.874 and 0.999 (mean = 0.982) in Zn-contaminated soils. Log IC50 values were estimated from the dose-response curves obtained for each concentration of metal, soil and antibiotic added (Figures 2–4). The mean log IC50 values corresponding to the uncontaminated control (0 mg kg−1 of Ni and Zn) after the addition of the tetracycline antibiotics to the bacterial suspension were 0.76 for TC, 0.78 for OTC and 0.41 for CTC, indicating that CTC is the most toxic among them, while TC and OTC show similar values. This sequence of toxicities is similar to that found in previous works, where CTC >> OTC ≥ TC (Santás-Miguel et al., 2020c; Santás-Miguel et al., 2020d).
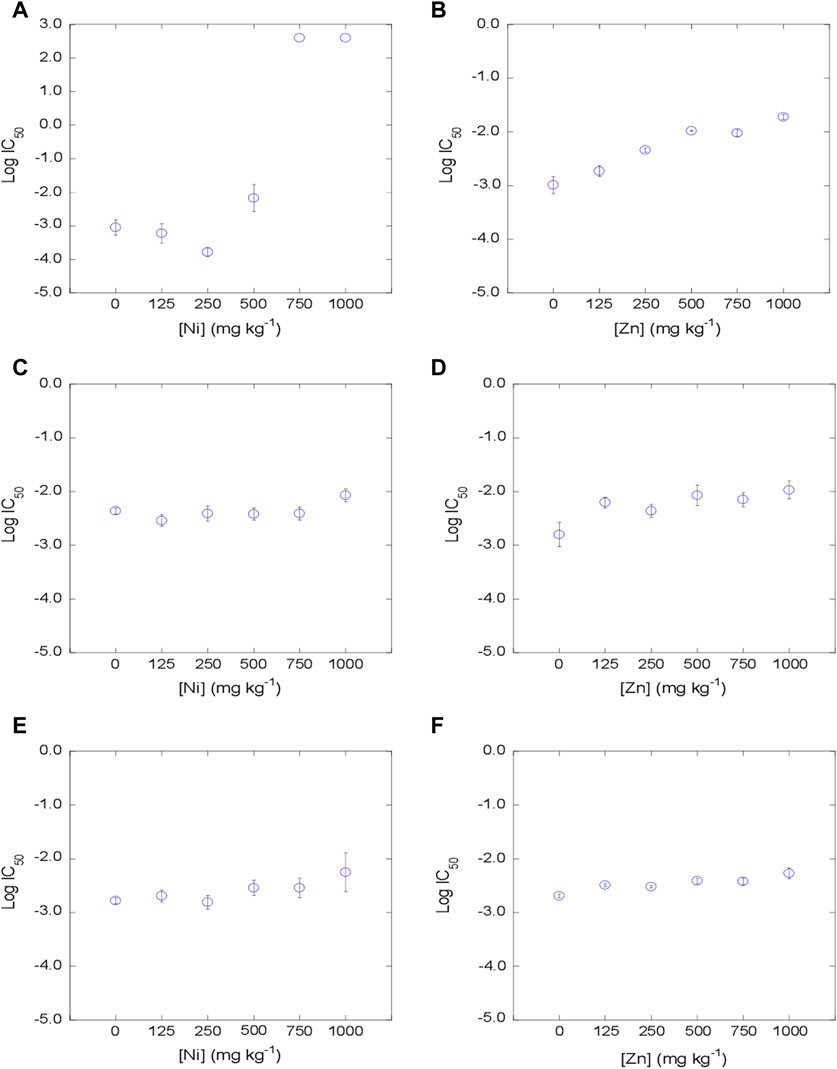
FIGURE 2. Evolution of bacterial community tolerance to TC (as log IC50), for increasing Ni and Zn concentration in the soil (0, 125, 250, 500, 750 and 1,000 mg kg−1). (A, B): soil 1; (C, D): soil 2, (E, F): soil 3.
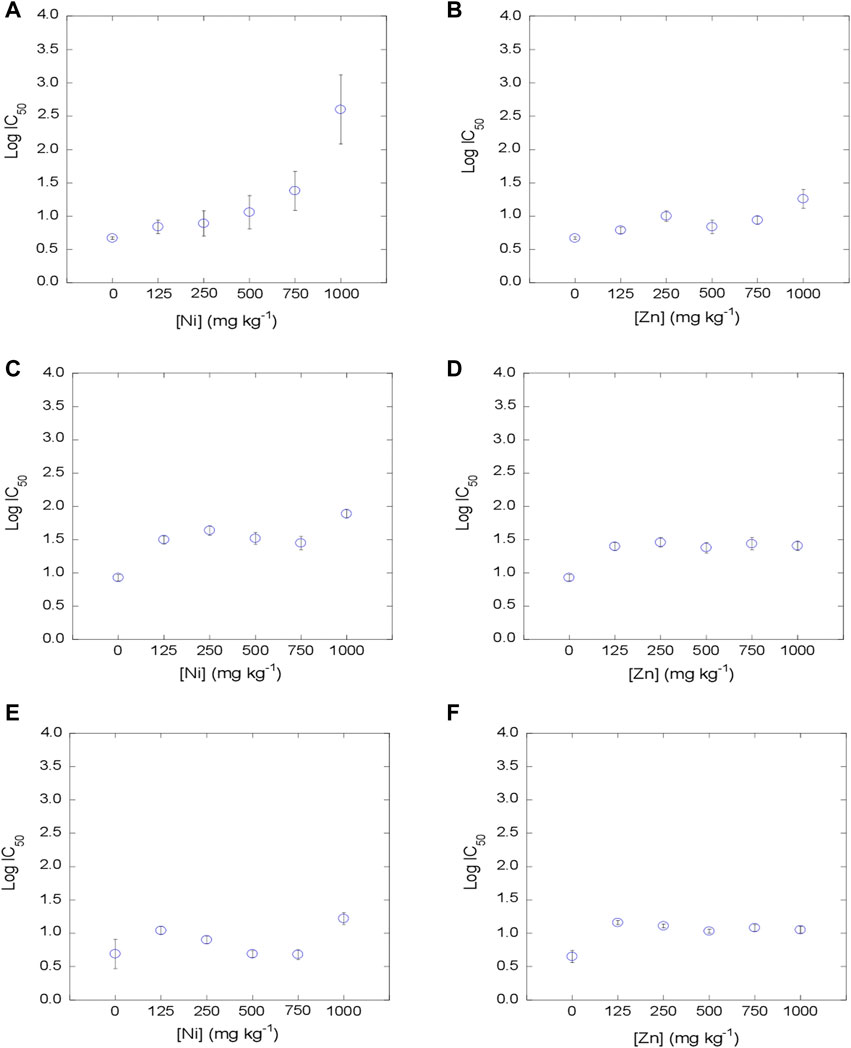
FIGURE 3. Evolution of bacterial community tolerance to OTC (as log IC50), for increasing Ni and Zn concentration in the soil (0, 125, 250, 500, 750 and 1,000 mg kg−1). (A, B): soil 1; (C, D): soil 2, (E, F): soil 3.
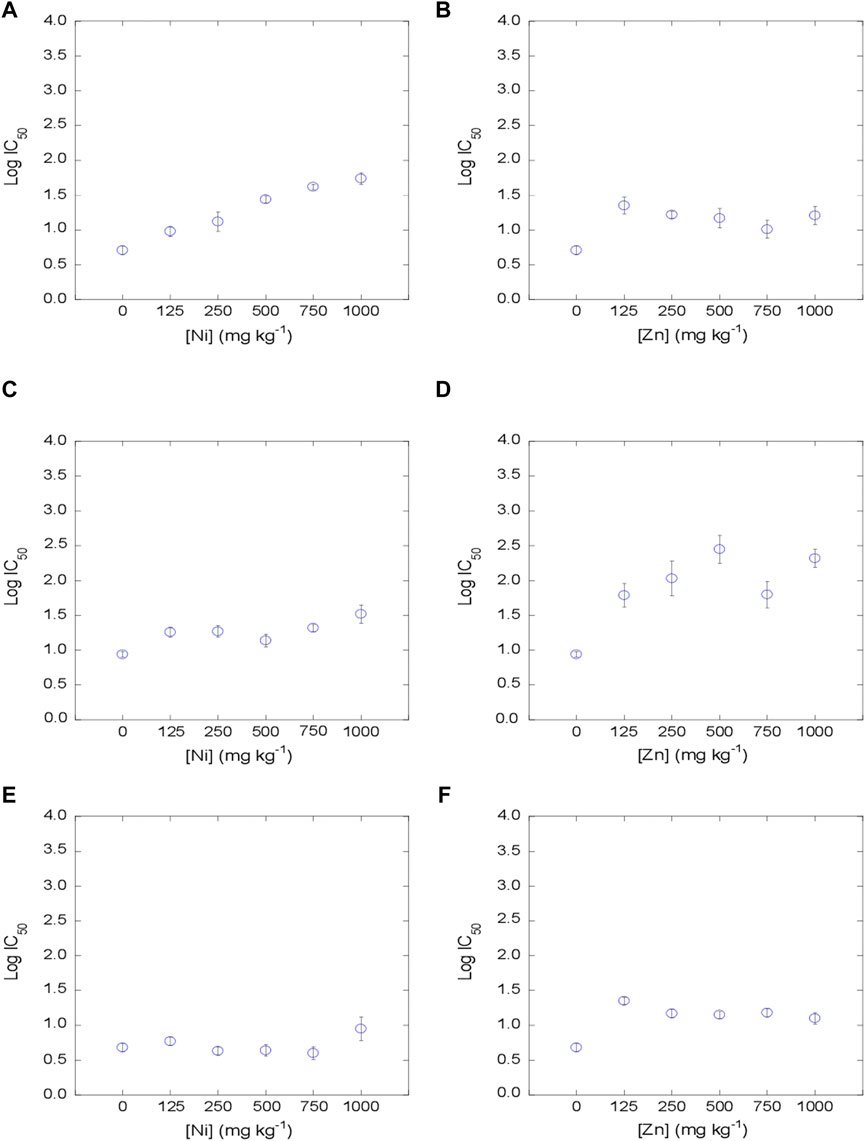
FIGURE 4. Evolution of bacterial community tolerance to CTC (as log IC50), for increasing Ni and Zn concentration in the soil (0, 125, 250, 500, 750 and 1,000 mg kg−1). (A, B): soil 1; (C, D): soil 2, (E, F): soil 3.
The co-tolerance increases obtained after exposure of the bacterial suspension to TC, OTC and CTC showed an identical behavior within these tetracycline antibiotics. However, it was dependent on the heavy metal tested (Ni or Zn). On the one hand, the tolerance increases of TC, OTC and CTC in soils contaminated with Ni were evidenced at concentrations ≥125 mg kg−1 in soil 1 (C = 1.1%) and 2 (C = 5.3%), whereas in soil 3, with a very high carbon content (C = 10.9%), increases in co-tolerance to tetracycline antibiotics took place at very high concentrations (≥1,000 mg kg−1). Regarding soils contaminated with different concentrations of Zn, co-tolerance increases were observed for TC, OTC and CTC at the lowest concentration tested of ≥125 mg kg−1 for the 3 soils. This behavior shown for tetracycline antibiotics is similar to that observed for heavy metals in the previous section, where Ni tolerance increases showed a slight dependence on soil carbon content, while Zn tolerance increases were independent of the soil carbon content. Therefore, the evidence from studies suggests that bacterial communities that have been previously exposed to heavy metals may also develop co-tolerance to antibiotics. There are several studies that show that environmental contamination with heavy metals shows a high correlation with antibiotic resistance genes (ARGs) (Ji et al., 2012; Pal et al., 2015; Chen et al., 2019; Lu et al., 2020). Lu et al. (2020) studied the abundance of heavy metals, antibiotics and ARGs in sediments from China with organic matter values between 89 and 120 g kg-1. These authors found that there is a positive correlation between most of the tetracycline ARGs analyzed (tetC, tetG, tetO, tetE) and the presence of Zn in the sediments. However, in the case of Ni, no correlations were found with most of the tetracycline ARGs studied (only with tetA and tetQ). However, there are various mechanisms that can participate in the acquisition of resistance to heavy metals and antibiotics, such as co-resistance, cross-resistance and co-regulated resitant.
As previously commented, many studies show that there are increases in co-tolerance to antibiotics in the presence of different heavy metals from a functional and genetic point of view (Berg et al., 2010; Knapp et al., 2011; Hu et al., 2017; Knapp et al., 2017; Song et al., 2017; Xu et al., 2017; Nguyen et al., 2019; Santás-Miguel et al., 2020b; Zhong et al., 2021; Santás-Miguel et al., 2022). But rather few studies have focused on the concentrations of heavy metals present in soils triggering the co-tolerance of soil bacterial communities to antibiotics and, more specifically, to tetracycline antibiotics. In this regard, Santás-Miguel et al. (2022) observed increases in co-tolerance to tetracycline antibiotics at 1,000 mg kg−1 of 7 heavy metals separately added to the soil, including Ni and Zn among them. Song et al. (2017) observed increases in co-tolerance to tetracycline in a soil sample (%C = 1.4) contaminated with Zn at concentrations ≥264 mg kg−1. However, Zhong et al. (2021) did not observe an increase in co-tolerance to TC in soils contaminated with different concentrations of Zn (33–3,811 mg kg−1). As for Ni, there are very few studies dealing with increases in co-tolerance to tetracycline antibiotics. Hu et al. (2017) observed an increase in antibiotic resistance genes (ARGs) at Ni concentrations in soil ≥100 mg kg−1, concentrations similar to those observed in the present study for soils with a carbon content ≤5.3% (soil 1 and 2). Therefore, although the effect of heavy metals on soil bacterial communities is a widely studied topic, additional research should be carried out in the future, to be added to the current investigation, to shed further light on what are the concentrations of different heavy metals from which there is a risk of increased co-tolerance to a vast variety of antibiotics. This environmental problem must be treated as a priority to recognize which are the soils with potential risk of presenting bacterial communities resistant to antibiotics and thus avoid the dissemination of antibiotic resistance genes in the environment.
Conclusion
In the present study it was observed that, for the 3 agricultural soils studied, Zn presented higher toxicity than Ni on soil bacterial communities. In addition, the soils contaminated with different concentrations of Ni and Zn showed increased tolerance to both metals, with a slight dependence on the soil carbon content in the case of Ni. However, for Zn these increments in tolerance were independent of the soil carbon content. In addition, the samples contaminated with Ni showed increases in co-tolerance from low concentrations of these heavy metals (≥125 mg kg−1) for tetracycline antibiotics (TC, OTC and CTC) for soils with carbon content ≤5.3%, whereas, for soil with a high carbon content (C = 10.9%), co-tolerance increases occur at concentrations ≥1,000 mg kg−1. On the other hand, the samples contaminated with Zn showed increases in co-tolerance from low concentrations of this metal (≥125 mg kg−1) for the 3 tetracycline antibiotics. As a suggestion for future research, the study of the interactions between different contaminants present in agricultural soils and the selection pressure they exert on soil bacterial communities should be addressed in more depth. The acquisition of this knowledge will allow the implementation of more appropriate agricultural soil management practices, such as the improvement of high-carbon amendments to reduce the bioavailability of these elements and thus reduce the risk to human and environmental health that contaminated soils show.
Data Availability Statement
The raw data supporting the conclusion of this article will be made available by the authors, without undue reservation.
Author contributions
Conceptualization; MA-E, EÁ-R, DF-C, AN-D, MD-R; Methodology: MA-E, EÁ-R, DF-C, AN-D, MD-R; Software: LR-G, VS-M; Data curation: MA-E, EÁ-R, DF-C, MD-R; Writing—Original draft preparation: VS-M, MA-E, DF-C, MD-R; Visualization: MA-E, EÁ-R, DF-C, AN-D, MD-R, LR-G, VS-M; Investigation: LR-G, VS-M; Supervision: MA-E, EÁ-R, DF-C, AN-D, MD-R; Validation: MA-E, EÁ-R, DF-C, AN-D, MD-R; Writing—Reviewing and Editing: MD-R, AN-D.
Funding
This study has been funded by the Spanish Ministry of Economy and Competitiveness through the projects CGL 2015-67333-C2-1-R and -2-R (FEDER Funds) and by Xunta de Galicia via BV1 research group (ED431C 2017/62-GRC). F-CD holds a Ramón y Cajal contract (RYC-2016-20411) financed by the Spanish Ministry of Economy, Industry and Competitiveness. S-MV Santás Miguel holds a posdoctoral fellowship (ED481B-2022-081) financed by Xunta de Galicia. A-RL hold a pre-doctoral fellowship (ED481A 2021/309) financed by Xunta de Galicia.
Conflict of Interest
The authors declare that the research was conducted in the absence of any commercial or financial relationships that could be construed as a potential conflict of interest.
Supplementary Material
The Supplementary Material for this article can be found online at: https://www.frontierspartnerships.org/articles/10.3389/sjss.2023.10799/full#supplementary-material
References
Abdu, N., Abdullahi, A. A., and Abdulkadir, A. (2017). Heavy Metals and Soil Microbes. Environ. Chem. Lett. 15 (1), 65–84. doi:10.1007/s10311-016-0587-x
Almås, Å. R., Bakken, L. R., and Mulder, J. (2004). Changes in Tolerance of Soil Microbial Communities in Zn and Cd Contaminated Soils. Soil Biol. biochem. 36 (5), 805–813. doi:10.1016/j.soilbio.2004.01.010
Bååth, E. (1989). Effects of Heavy Metals in Soil on Microbial Processes and Populations (A Review). Water Air Soil Pollut. 47 (3), 335–379. doi:10.1007/BF00279331
Berg, J., Thorsen, M. K., Holm, P. E., Jensen, J., Nybroe, O., and Brandt, K. K. (2010). Cu Exposure under Field Conditions Coselects for Antibiotic Resistance as Determined by a Novel Cultivationindependent Bacterial Community Tolerance Assay. Environ. Sci. Technol. 44, 8724–8728. doi:10.1021/es101798r
Blanck, H. (2002). A Critical Review of Procedures and Approaches Used for Assessing Pollution-Induced Community Tolerance (PICT) in Biotic Communities. Hum. Ecol. Risk Assess. 8 (5), 1003–1034. doi:10.1080/1080-700291905792
Brookes, P. C. (1995). The Use of Microbial Parameters in Monitoring Soil Pollution by Heavy Metals. Biol. Fertil. Soils. 19 (4), 269–279. doi:10.1007/BF00336094
Caban, J. R., Kuppusamy, S., Kim, J. H., Yoon, Y. E., Kim, S. Y., and Lee, Y. B. (2018). Green Manure Amendment Enhances Microbial Activity and Diversity in Antibiotic Contaminated Soil. Appl. Soil Ecol. 129, 72–76. doi:10.1016/j.apsoil.2018.04.013
Chen, J., Li, J., Zhang, H., Shi, W., and Liu, Y. (2019). Bacterial Heavy-Metal and Antibiotic Resistance Genes in a Copper Tailing Dam Area in Northern China. Front. Microbiol. 10, 1916. doi:10.3389/fmicb.2019.01916
Conde-Cid, M., Álvarez-Esmorís, C., Paradelo-Núñez, R., Nóvoa-Muñoz, J. C., Arias- Estévez, M., Álvarez-Rodríguez, E., et al. (2018). Occurrence of Tetracyclines and Sulfonamides in Manures, Agricultural Soils and Crops from Different Areas in Galicia (NW Spain). J. Clean. Prod. 197, 491–500. doi:10.1016/j.jclepro.2018.06.217
Davies, J. (1994). Inactivation of Antibiotics and the Dissemination of Resistance Genes. Science 264 (5157), 375–382. doi:10.1126/science.8153624
Davis, M. R., Zhao, F. J., and McGrath, S. P. (2004). Pollution-induced Community Tolerance of Soil Microbes in Response to a Zinc Gradient. Environ. Toxicol. Chem. 23 (11), 2665–2672. doi:10.1897/03-645
Diaz-Ravina, M., and Baath, E. (1996). Development of Metal Tolerance in Soil Bacterial Communities Exposed to Experimentally Increased Metal Levels. Appl. Environ. Microbiol. 62 (8), 2970–2977. doi:10.1128/aem.62.8.2970-2977.1996
Díaz-Raviña, M., Bååth, E., and Frostegård, Å. (1994). Multiple Heavy Metal Tolerance of Soil Bacterial Communities and its Measurement by a Thymidine Incorporation Technique. Appl. Environ. Microbiol. 60 (7), 2238–2247. doi:10.1128/aem.60.7.2238-2247.1994
Dıaz-Ravina, M., and Bååth, E. (2001). Response of Soil Bacterial Communities Pre-exposed to Different Metals and Reinoculated in an Unpolluted Soil. Soil Biol. biochem. 33 (2), 241–248. doi:10.1016/S0038-0717(00)00136-X
Dickinson, A. W., Power, A., Hansen, M. G., Brandt, K. K., Piliposian, G., Appleby, P., et al. (2019). Heavy Metal Pollution and Co-selection for Antibiotic Resistance: A Microbial Palaeontology Approach. Environ. Int. 132, 105117. doi:10.1016/j.envint.2019.105117
Duxbury, T., and Bicknell, B. (1983). Metal-tolerant Bacterial Populations from Natural and Metal-Polluted Soils. Soil Biol. biochem. 15 (3), 243–250. doi:10.1016/0038-0717(83)90066-4
EC (2006). “European Commission Regulation EC 1881/2006. Setting Maximum Levels for Certain Contaminants in Foodstuffs”,” in Official Journal of the European Union EC 1881/2006. (20.12.2006), L364/365-L364/324.
European Medicines Agency (2016). “European Surveillance of Veterinary Antimicrobial Consumption,” in Sales of Veterinary Antimicrobial Agents in 29 European Countries in 2014. EMA/61769/2016.
Fernández-Calviño, D., and Bååth, E. (2013). Co-selection for Antibiotic Tolerance in Cu-Polluted Soil Is Detected at Higher Cu-Concentrations Than Increased Cu-Tolerance. Soil Biol. biochem. 57, 953–956. doi:10.1016/j.soilbio.2012.08.017
Giller, K. E., Witter, E., and McGrath, S. P. (1999). Assessing Risks of Heavy Metal Toxicity in Agricultural Soils: Do Microbes Matter? Hum. Ecol. Risk Assess. 5 (4), 683–689. doi:10.1080/10807039.1999.9657732
Giller, K. E., Witter, E., and McGrath, S. P. (2009). Heavy Metals and Soil Microbes. Soil Biol. biochem. 41 (10), 2031–2037. doi:10.1016/j.soilbio.2009.04.026
Giller, K. E., Witter, E., and Mcgrath, S. P. (1998). Toxicity of Heavy Metals to Microorganisms and Microbial Processes in Agricultural Soils: a Review. Soil Biol. biochem. 30 (10-11), 1389–1414. doi:10.1016/S0038-0717(97)00270-8
Gluskoter, H. J. (1977). Trace Elements in Coal: Occurrence and Distribution. Urbana, IL. Circular no. 499.
Hamscher, G., Sczesny, S., Höper, H., and Nau, H. (2002). Determination of Persistent Tetracycline Residues in Soil Fertilized with Liquid Manure by High-Performance Liquid Chromatography with Electrospray Ionization Tandem Mass Spectrometry. Anal. Chem. 74 (7), 1509–1518. doi:10.1021/ac015588m
Hattori, H. (1992). Influence of Heavy Metals on Soil Microbial Activities. Soil Sci. Plant Nutr. 38 (1), 93–100. doi:10.1080/00380768.1992.10416956
He, Z., Endale, D. M., Schomberg, H. H., and Jenkins, M. B. (2009). Total Phosphorus, Zinc, Copper, and Manganese Concentrations in Cecil Soil through 10 Years of Poultry Litter Application. Soil Sci. 174 (12), 687–695. doi:10.1097/SS.0b013e3181c30821
Hejna, M., Gottardo, D., Baldi, A., Dell’Orto, V., Cheli, F., Zaninelli, M., et al. (2018). Review: Nutritional Ecology of Heavy Metals. Animal 12 (10), 2156–2170. doi:10.1017/S175173111700355X
Hu, H. W., Wang, J. T., Li, J., Shi, X. Z., Ma, Y. B., Chen, D., et al. (2017). Long-term Nickel Contamination Increases the Occurrence of Antibiotic Resistance Genes in Agricultural Soils. Environ. Sci. Technol. 51 (2), 790–800. doi:10.1021/acs.est.6b03383
Ji, X., Shen, Q., Liu, F., Ma, J., Xu, G., Wang, Y., et al. (2012). Antibiotic Resistance Gene Abundances Associated with Antibiotics and Heavy Metals in Animal Manures and Agricultural Soils Adjacent to Feedlots in Shanghai; China. J. Hazard. Mat. 235, 178–185. doi:10.1016/j.jhazmat.2012.07.040
Knapp, C. W., Callan, A. C., Aitken, B., Shearn, R., Koenders, A., and Hinwood, A. (2017). Relationship between Antibiotic Resistance Genes and Metals in Residential Soil Samples from Western Australia. Environ. Sci. Pollut. Res. 24 (3), 2484–2494. doi:10.1007/s11356-016-7997-y
Knapp, C. W., Dolfing, J., Ehlert, P. A., and Graham, D. W. (2010). Evidence of Increasing Antibiotic Resistance Gene Abundances in Archived Soils since 1940. Environ. Sci. Technol. 44 (2), 580–587. doi:10.1021/es901221x
Knapp, C. W., McCluskey, S. M., Singh, B. K., Campbell, C. D., Hudson, G., and Graham, D. W. (2011). Antibiotic Resistance Gene Abundances Correlate with Metal and Geochemical Conditions in Archived Scottish Soils. PloS One 6 (11), e27300. doi:10.1371/journal.pone.0027300
Lu, L., Liu, J., Li, Z., Zou, X., Guo, J., Liu, Z., et al. (2020). Antibiotic Resistance Gene Abundances Associated with Heavy Metals and Antibiotics in the Sediments of Changshou Lake in the Three Gorges Reservoir Area, China. Ecol. Indic. 113, 106275. doi:10.1016/j.ecolind.2020.106275
Macías, F., and Calvo, R. (2008). “Niveles genéricos de referencia de metales pesados y otroselementos traza en suelos de Galicia (Reference values for heavy metals and other traceelements in soils in Galicia),” in Consellería de Medio Ambiente e Desenvolvemento Sostible (Santiago de Compostela, Spain: Xunta de Galicia), 229.
Meisner, A., Bååth, E., and Rousk, J. (2013). Microbial Growth Responses Upon Rewetting Soil Dried for Four Days or One Year. Soil Biol. Biochem. 66, 188–192. doi:10.1016/j.soilbio.2013.07.014
Milne, C. J., Kinniburgh, D. G., Van Riemsdijk, W. H., and Tipping, E. (2003). Generic NICA—Donnan Model Parameters for Metal-Ion Binding by Humic Substances. Environ. Sci. Technol. 37 (5), 958–971. doi:10.1021/es0258879
Nguyen, C. C., Hugie, C. N., Kile, M. L., and Navab-Daneshmand, T. (2019). Association between Heavy Metals and Antibiotic-Resistant Human Pathogens in Environmental Reservoirs: a Review. Front. Environ. Sci. Eng. 13 (3), 46–17. doi:10.1007/s11783-019-1129-0
Nies, D. H. (1999). Microbial Heavy-Metal Resistance. Appl. Microbiol. Biotechnol. 51 (6), 730–750. doi:10.1007/s002530051457
Oyetibo, G. O., Ilori, M. O., Adebusoye, S. A., Obayori, O. S., and Amund, O. O. (2010). Bacteria with Dual Resistance to Elevated Concentrations of Heavy Metals and Antibiotics in Nigerian Contaminated Systems. Environ. Monit. Assess. 168 (1), 305–314. doi:10.1007/s10661-009-1114-3
Pal, C., Bengtsson-Palme, J., Kristiansson, E., and Larsson, D. G. (2015). Co-occurrence of Resistance Genes to Antibiotics, Biocides and Metals Reveals Novel Insights into Their Co-selection Potential. BMC genomics 16 (1), 964–1014. doi:10.1186/s12864-015-2153-5
Piccolo, A. (1989). Reactivity of Added Humic Substances towards Plant Available Heavy Metals in Soils. Sci. Total Environ. 81, 607–614. doi:10.1016/0048-9697(89)90169-1
Refaey, Y., Jansen, B., El-Shater, A. H., El-Haddad, A. A., and Kalbitz, K. (2014). The Role of Dissolved Organic Matter in Adsorbing Heavy Metals in Clay-Rich Soils. Vadose Zone J. 13 (7), vzj2014010009. doi:10.2136/vzj2014.01.0009
Rodríguez-López, L., Santás-Miguel, V., Núñez-Delgado, A., Álvarez-Rodríguez, E., Pérez-Rodríguez, P., and Arias-Estévez, M. (2022). Influence of pH, Humic Acids, and Salts on the Dissipation of Amoxicillin and Azithromycin under Simulated Sunlight. Span. J. Soil Sci. 12. doi:10.3389/sjss.2022.10438
Rule, J. H. (1999). Trace Metal Cation Adsorption in Soils: Selective Chemical Extractions and Biological Availability. Stud. Surf. Sci. Catal. 120, 319–349. doi:10.1016/S0167-2991(99)80364-4
Santás-Miguel, V., Arias-Estévez, M., Díaz-Raviña, M., Fernández-Sanjurjo, M. J., Álvarez-Rodríguez, E., Núñez-Delgado, A., et al. (2020b). Bacterial Community Tolerance to Tetracycline Antibiotics in Cu Polluted Soils. Agronomy 10, 1220. doi:10.3390/agronomy10091220
Santás-Miguel, V., Arias-Estévez, M., Díaz-Raviña, M., Fernández-Sanjurjo, M. J., Álvarez-Rodríguez, E., Núñez-Delgado, A., et al. (2020d). Effect of Oxytetracycline and Chlortetracycline on Bacterial Community Growth in Agricultural Soils. Agronomy 10 (7), 1011. doi:10.3390/agronomy10071011
Santás-Miguel, V., Arias-Estévez, M., Díaz-Raviña, M., Fernández-Sanjurjo, M. J., Álvarez-Rodríguez, E., Núñez-Delgado, A., et al. (2020c). Interactions between Soil Properties and Tetracycline Toxicity Affecting to Bacterial Community Growth in Agricultural Soil. Appl. Soil Ecol. 147, 103437. doi:10.1016/j.apsoil.2019.103437
Santás-Miguel, V., Díaz-Raviña, M., Martín, A., García-Campos, E., Barreiro, A., Núñez-Delgado, A., et al. (2020a). Medium-term Influence of Tetracyclines on Total and Specific Microbial Biomass in Cultivated Soils of Galicia (NW Spain). Span. J. Soil Sci. 10, 217–232. doi:10.3232/SJSS.2020.V10.N3.05
Santás-Miguel, V., Núñez-Delgado, A., Álvarez-Rodríguez, E., Díaz-Raviña, M., Arias-Estévez, M., and Fernández-Calviño, D. (2022). Tolerance of Soil Bacterial Community to Tetracycline Antibiotics Induced by as, Cd, Zn, Cu, Ni, Cr, and Pb Pollution. SOIL 8 (1), 437–449. doi:10.5194/soil-8-437-2022
Sapkota, A., Sapkota, A. R., Kucharski, M., Burke, J., McKenzie, S., Walker, P., et al. (2008). Aquaculture Practices and Potential Human Health Risks: Current Knowledge and Future Priorities. Environ. Int. 34 (8), 1215–1226. doi:10.1016/j.envint.2008.04.009
Sarmah, A. K., Meyer, M. T., and Boxall, A. B. (2006). A Global Perspective on the Use, Sales, Exposure Pathways, Occurrence, Fate and Effects of Veterinary Antibiotics (VAs) in the Environment. Chemosphere 65 (5), 725–759. doi:10.1016/j.chemosphere.2006.03.026
Sauvé, S., Manna, S., Turmel, M. C., Roy, A. G., and Courchesne, F. (2003). Solid− Solution Partitioning of Cd, Cu, Ni, Pb, and Zn in the Organic Horizons of a Forest Soil. Environ. Sci. Technol. 37 (22), 5191–5196. doi:10.1021/es030059g
Sebaugh, J. L. (2011). Guidelines for Accurate EC50/IC50 Estimation. Pharm. Stat. 10 (2), 128–134. doi:10.1002/pst.426
Seiler, C., and Berendonk, T. U. (2012). Heavy Metal Driven Co-selection of Antibiotic Resistance in Soil and Water Bodies Impacted by Agriculture and Aquaculture. Front. Microbiol. 3, 399. doi:10.3389/fmicb.2012.00399
Serwecińska, L. (2020). Antimicrobials and Antibiotic-Resistant Bacteria: a Risk to the Environment and to Public Health. Water 12 (12), 3313. doi:10.3390/w12123313
Song, J., Rensing, C., Holm, P. E., Virta, M., and Brandt, K. K. (2017). Comparison of Metals and Tetracycline as Selective Agents for Development of Tetracycline Resistant Bacterial Communities in Agricultural Soil. Environ. Sci. Technol. 51 (5), 3040–3047. doi:10.1021/acs.est.6b05342
Spark, K. M., Wells, J. D., and Johnson, B. B. (1997). The Interaction of a Humic Acid with Heavy Metals. Soil Res. 35 (1), 89–102. doi:10.1071/S96008
Stefanowicz, A. M., Niklińska, M., and Laskowski, R. (2009). Pollution-induced Tolerance of Soil Bacterial Communities in Meadow and Forest Ecosystems Polluted with Heavy Metals. Eur. J. Soil Biol. 45 (4), 363–369. doi:10.1016/j.ejsobi.2009.05.005
Swift, M. J. (1994). “Maintaining the Biological Status of Soil: a Key to Sustainable Land Management,” in Soil Resilience and Sustainable Land Use. Editors D. J. Greenland,, and I. Szabolcs (Wallingford: CAB), 33–39.
Thiele-Bruhn, S. (2005). Microbial Inhibition by Pharmaceutical Antibiotics in Different Soils—Dose-response Relations Determined with the Iron (III) Reduction Test. Environ. Toxicol. Chem. 24 (4), 869–876. doi:10.1897/04-166R.1
Toth, J. D., Feng, Y., and Dou, Z. (2011). Veterinary Antibiotics at Environmentally Relevant Concentrations Inhibit Soil Iron Reduction and Nitrification. Soil Biol. biochem. 43 (12), 2470–2472. doi:10.1016/j.soilbio.2011.09.004
Urra, J., Alkorta, I., Lanzén, A., Mijangros, I., and Garbisu, C. (2019). The Application of Fresh and Composted Horse and Chicken Manure Affects Soil Quality, Microbial Composition and Antibiotic Resistance. Appl. Soil Ecol. 135, 73–84. doi:10.1016/j.apsoil.2018.11.005
Wu, L., Pan, X., Chen, L., Huang, Y., Teng, Y., Luo, Y., et al. (2013). Occurrence and Distribution of Heavy Metals and Tetracyclines in Agricultural Soils after Typical Land Use Change in East China. Environ. Sci. Pollut. Res. 20, 8342–8354. doi:10.1007/s11356-013-1532-1
Xu, Y., Xu, J., Mao, D., and Luo, Y. (2017). Effect of the Selective Pressure of Sub-lethal Level of Heavy Metals on the Fate and Distribution of ARGs in the Catchment Scale. Environ. Pollut. 220, 900–908. doi:10.1016/j.envpol.2016.10.074
Zhang, F., Li, Y., Yang, M., and Li, W. (2012). Content of Heavy Metals in Animal Feeds and Manures from Farms of Different Scales in Northeast China. Int. J. Environ. Res. Public Health. 9 (8), 2658–2668. doi:10.3390/ijerph9082658
Keywords: antibiotics, bacterial growth, heavy metals, leucine incorporation method, PICT
Citation: Santás-Miguel V, Rodríguez-González L, Núñez-Delgado A, Álvarez-Rodríguez E, Díaz-Raviña M, Arias-Estévez M and Fernández-Calviño D (2023) Soil Bacterial Community Tolerance to Three Tetracycline Antibiotics Induced by Ni and Zn. Span. J. Soil Sci. 13:10799. doi: 10.3389/sjss.2023.10799
Received: 26 July 2022; Accepted: 11 January 2023;
Published: 19 January 2023.
Edited by:
Xose Luis Otero, Scientific coordinator of REBUSC (Network of Biological Stations of the University of Santiago de Compostela), SpainCopyright © 2023 Santás-Miguel, Rodríguez-González, Núñez-Delgado, Álvarez-Rodríguez, Díaz-Raviña, Arias-Estévez and Fernández-Calviño. This is an open-access article distributed under the terms of the Creative Commons Attribution License (CC BY). The use, distribution or reproduction in other forums is permitted, provided the original author(s) and the copyright owner(s) are credited and that the original publication in this journal is cited, in accordance with accepted academic practice. No use, distribution or reproduction is permitted which does not comply with these terms.
*Correspondence: Vanesa Santás-Miguel, dnNhbnRhc0B1dmlnby5lcw==