- 1Laboratorio de Genotoxicología, Unidad de Investigación en Ambiente y Salud, Universidad Autónoma de Occidente, Los Mochis, Sinaloa, Mexico
- 2Centro de Investigación en Ciencias Biológicas, Universidad Autónoma de Tlaxcala, San Felipe Ixtacuixtla, Tlaxcala, México
- 3Centro de Investigación en Alimentación y Desarrollo, A.C. Unidad Culiacán, Culiacán, Sinaloa, Mexico
Using microbial cells for bioremediation requires evaluating suitable inoculation techniques and their effects. This study applied liquid and encapsulated in alginate beads inocula of A. vinelandii in agricultural soil to evaluate chlorpyrifos (CP) degradation and its impact on cytotoxic and genotoxic effects. Allium sativum cells and Eisenia foetida organisms were used as biomarkers for toxicological evaluations. Changes in the mitotic index and nuclear abnormalities in A. sativum cells were used for toxicity determinations. The percentage survival of E. foetida was calculated. Ultra-high-performance liquid chromatography was used to detect CP. The initial CP concentration (250 mg/kg) decreased by 92% when inoculated with liquid A. vinelandii and by 82% with A. vinelandii encapsulated after 14 d. A 60% decrease in cytotoxic and genotoxic damage to A. sativum cells was detected in treatments inoculated with A. vinelandii. The survival rate of E. foetida was improved by 33% when inoculated with free A. vinelandii compared to contaminated soil. Encapsulation as an inoculation strategy extended the viability of A. vinelandii compared to free inoculation. Both free and encapsulated inocula of A. vinelandii effectively degrade CP in soil and decrease its toxic effects. This study contributed by identifying sustainable agricultural alternatives for the inoculation and bioremediation of agricultural soils.
Introduction
Chlorpyrifos (O, O-diethylO-3,5,6-trichloro-2-pyridyl-phosphorothioate, CP) is among the most widely used pesticides worldwide and a broad-spectrum organophosphate insecticide that produces multiple adverse effects on the environment and human health (Norén et al., 2020; Zhang et al., 2021). CP acts by inhibiting the enzyme acetylcholinesterase (AChE), affecting the nervous system of insects, mammals, fish, and humans (World Health Organization, 2015; Malla et al., 2023). CP is associated with neurological disorders, cancer, hormonal disruption, kidney damage and reproductive problems (Huang et al., 2021; Aung et al., 2022; Sun et al., 2023). Similarly, damage to other organisms, such as pollinators, annelids, and soil microbiota has been reported (Wang e al., 2020; Ju et al., 2023; Cheng et al., 2023).
Given the toxicity of CP, in 2021, some countries implemented measures to ban it, like the USA and the European Union (Hites, 2021; Pechen and Venturino, 2021). Notably, an important initiative by the European Union involves the proposal to include CP in Annex A of the Stockholm Convention, which lists the most hazardous organic substances worldwide (EPA, Environmental Protection Agency, 2022; United Nations Environment Programme, 2023). However, CP continues to be widely used without monitoring or usage restriction measures for either CP or its metabolites (Ruiz-Arias et al., 2023). Consequently, developing and implementing strategies for detecting and mitigating CP induced damage in different environmental matrices such as food, air, water, sediments, and soil are necessary.
The soil is a primary entry route for pesticides into the environment and trophic chains. CP is usually applied repeatedly in a crop cycle and in concentrations higher than recommended by the manufacturer (250 mg/ha). It can enter the soil by several routes, mainly by direct application as a preventive treatment, in solid and liquid form, drench or foliar application; by spray drift in aerial applications by light aircraft or drone to agricultural fields; by treatment of gardens and airstrips, tank washing, and through agricultural wastewater (Huang et al., 2021; Bose et al., 2021; Elzakey et al., 2023).
In soil, macro, meso, microfauna, and microbial communities, that are essential for nutrient management, availability, and biodegradation, are severely affected by CP and other pesticides (Zhu et al., 2020; Hou et al., 2023). Consequently, the symbiotic and ecological functions that facilitate plant growth are directly and indirectly damaged (Kisvarga et al., 2023). To reduce the negative impact, the biodegradation of pesticides like CP in agricultural soils can be achieved using beneficial microorganisms capable of tolerating or eliminating contaminants by adapting to adverse conditions. However, due to the chemical properties of CP, its frequent application, and environmental variability, the parent molecule and its metabolites are challenging to remove (EPA, Environmental Protection Agency, 2022).
CP is a compound derived from thiophosphoric acid with chemical formula C9H11Cl3NO3PS and molecular weight of 350.6 g/mol (Figure 1). It has low solubility in water (1.4 mg/L at 25°C) and high retention in soil (360–31,000 L/kg) (Bose et al., 2021). It is generally estimated that the half-life of CP in the environment can be 100–386 d (United Nations Environment Programme, 2023). However, it may have variable ranges in different soil types (De Bernardi et al., 2022), so the estimate is discrete in terms of acute toxicity risks and the wide variability of environmental conditions (Foong et al., 2020). CP is usually resistant to hydrolysis at acidic pH, especially at low ambient temperatures and high organic matter content, which can extend its permanence up to years (Bose et al., 2021).
CP degradation pathways include abiotic (volatilization, oxidation, photodegradation) and biotic forms (biodegradation or mineralization, either individual or cometabolic). The latter is the most effective for their complete elimination. The biodegradation of CP has been tested employing efficient bacteria of the genera Pseudomonas, Bacillus, Cupriavidus, Ochrobactrum individually (Deng et al., 2015; Akbar and Sultan, 2016; Abraham and Silambarasan, 2016; Malla et al., 2023), with fungi of the genera Aspergillus, Byssochlamys, Ganoderma, Trichoderma (Jayaraman et al., 2012; Silambarasan and Abraham, 2014; Kumar et al., 2021; Bose et al., 2021); and in consortia consisting of different bacteria and fungi species (Khalid et al., 2018; Abraham and Silambarasan, 2018; Huang et al., 2021; Elzakey et al., 2023). Such studies have been mainly carried out through in vitro assays with varied degradation efficiencies. Most microorganisms show impairment and decreased degradation capacity at concentrations above 200 mg/L CP, and their efficiency usually declines in soil tests (Deng et al., 2015; Conde-Avila et al., 2021).
The microorganism’s ability to degrade CP is related to the production of enzymes such as chlorpyrifos hydrolase, organophosphorus hydrolase, phosphotriesterase, methyl parathion hydrolase, other metalloenzymes (Schenk et al., 2016), and laccases in the case of fungi (Kumar et al., 2021). The genes opdA, opdB, mpd, and cpd are strongly related to such metabolic capacity (Huang et al., 2021; Bose et al., 2021). The main CP degradation products are CP oxon (an even more toxic compound resulting from CP oxidation), O, Odiethyl thiophosphate (DETP), and 3,5,6-trichloro-2-pyridinol (TCP) (resulting from enzymatic cleavage of CP by hydrolysis). DETP, upon hydrolysis, yields phosphorothioic acid and ethanol. TCP is much more persistent and easier to translocate due to its solubility in water compared to the original molecule. TCP is an endocrine disruptor with a bactericidal effect, thanks to its structure with three chlorine atoms and an aromatic nitrogen ring, which most microorganisms cannot tolerate or degrade, which is a limiting step in the CP degradation pathway (Jabeen et al., 2015; Li et al., 2020; Bose et al., 2021).
Therefore, the diversity of organisms capable of fully and efficiently degrading both CP and TCP is limited, and many of its effects in agricultural soils still need to be better understood (Malla et al., 2023).
A current sustainable approach is to identify symbiotic, nutrient-fixing, and pesticide degrading microorganisms, alongside developing strategies to improve their viability and effectiveness, especially under stress conditions (Gomathy et al., 2021). A promising alternative is the use of plant growth-promoting rhizobacteria (PGPR), known for their contribution to plant growth and protection, potentially reducing or even replacing the need for chemical fertilizers and pesticides (Lewis, 2022). However, integrating PGPR into agricultural production faces several challenges, including their tolerance to contaminants in soil, variability in performance across different environments, biological interactions with native organisms, cultivation techniques, formulation processes, and overall effectiveness (Strobel et al., 2018). It has been identified that the most important challenges of effective inoculation occur in the first days (Bashan et al., 2014). Therefore, applying promising PGPR inoculation techniques and evaluating their efficacy in the degradation of toxic substances in soil is essential to generate efficient and sustainable alternatives (Conde-Avila et al., 2020; Gallego and Olivero-Verbel, 2021).
Among the PGPRs, the genus Azotobacter is an efficient free-living dinitrogen (N2) fixer (Apriliya and Mulyawan, 2022). Additionally, Azotobacter spp. produce polymers, phytohormones, antifungals, alkylresorcinols, and nutrient chelators (Vejan et al., 2016; Mankar, Sahay, and Gothawal, 2020; Bolaños-Dircio et al., 2022). Azotobacter spp. have also demonstrated tolerance and ability to degrade aromatic substances such as phenols (Revillas et al., 2000), pesticides (Chennappa, Sreenivasa, and Nagaraja, 2018; Mousa et al., 2021), hydrocarbons (Devianto et al., 2020) and sequestering heavy metals using exopolysaccharides (Hindersah et al., 2023). Laboratory have revealed that some Azotobacter spp. exhibit tolerance to CP, with varying effects on their metabolism and respiration depending on strain and medium conditions (Chennappa et al., 2019). In contrast, our research group has identified a highly tolerant and efficient strain of Azotobacter vinelandii (ATCC 12837) capable of mineralizing CP, i.e., 500 mg/L in 6 h under in vitro conditions, superior to degradation with other Azotobacter spp. and other microorganisms (Deng et al., 2015; Huang et al., 2021; Conde-Avila et al., 2021). Furthermore, we have applied an alginate encapsulation strategy that not only protects the cells but also enhances their ability to promote plant growth in soil (Conde-Avila et al., 2022). Encapsulation involves confining metabolically active cells within a non-toxic polymeric matrix to improve their establishment in field conditions, maintain their viability over an extended period and enable them to tolerate higher contaminant concentrations (Conde-Avila et al., 2020).
Despite the potential of PGPRs, the effectiveness of free and encapsulated A. vinelandii in the degradation of CP in agricultural soil in competition with a native environment and microbiota, as well as its effects on soil cytotoxicity and genotoxicity reduction, is unknown. Some studies have been conducted on the degradation of CP by bacteria or evaluations of toxicological damage and oxidative stress on non-target organisms like Allium cepa cells and earthworms (Santo et al., 2023; Ju et al., 2023; Cheng et al., 2023). However, few proposals have evaluated novel encapsulated formulations of efficient strains for CP bioremediation and the reduction of pesticide toxicological damage in agricultural soils.
The objective of this study was to evaluate the degradation of CP in soil using both free and encapsulated in alginate-Na beads A. vinelandii and to determine effects on cytotoxicity and genotoxicity using plant meristematic cells (Allium sativum) and earthworms (Eisenia foetida) as biomarkers of damage. Insights derived from this study contribute significantly to the monitoring and comprehension of pesticide toxicity effects, thereby promoting the amelioration of soil quality and the generation of sustainable agricultural alternatives.
MetHods
Strain Characteristics
The strain used in this study was A. vinelandii ATCC 12837. Cells were cryopreserved at 70°C in sterile 4% (v/v) glycerol solution. For both plate-based and in liquid culture propagation on, Burk-Sucrose (BS) medium was used with the following composition (g L1): sucrose 20, yeast extract (Difco™ BS, USA) 3, K2HPO4 0.66, KH2PO4 0.16, NaCl 0.2, MgSO4-7H2O 0.2, CaSO4 0.05, Na2MoO4-2H2O 0.0029, FeSO4-7H2O 0.027 and an initial pH of 7.
Obtaining the Liquid and Encapsulated Inoculum
A. vinelandii cells were grown at 28°C in 500 mL Erlenmeyer flasks with 100 mL of BS medium at 200 rpm until a concentration of 4 × 108 CFU/mL was reached. The biomass was collected by centrifugation at 1000 × g for 10 min, and the supernatant was discarded. The recovered biomass was mixed with sterile distilled water and used as liquid inoculum for subsequent experiments. To encapsulate A. vinelandii, the biomass pellet was mixed in a sterile 2% (w/v) alginate-Na solution. An aliquot of the alginate-Na and A. vinelandii suspension was added by drip to a sterile 0.1 M CaCl, forming 3–5 mm diameter beads.
Experimental Conditions
The treatments used in the experiment were: Control (No inoculum/no pesticide), Control + CP, Liquid inoculum, Liquid inoculum + CP, Encapsulated inoculum and Encapsulated inoculum + CP. A completely randomized design with four independent replicates (n = 4) per treatment was used. Sterile polyethylene pots of 10 cm diameter were used as experimental units and filled with 80 g of soil for treatment preparation. A commercial formulation of CP was used (Clorver 480® EC Versa Agrochemicals, Mexico) at a concentration of 250 mg of active ingredient per kg of soil. The following factors were considered in the experiment: 1) pesticide concentration (0 and 250 mg/kg of CP) and 2) type of A. vinelandii inoculum (liquid, encapsulated, and control without inoculum). Inoculums were adjusted to a cell density of 4 × 108 CFU/mL for both inoculated treatments. Soil inoculation was performed with 6 mL of A. vinelandii inoculum in a liquid solution (inoculum diluted in 54 mL of distilled water), and its equivalent encapsulated in alginate Na beads per experimental unit. A control treatment without inoculum was included, to which 60 mL of distilled water was applied. The experiment was conducted under controlled temperature (28°C) and soil moisture adjusted according to field capacity until 50%. During the experiment, soil samples were taken in triplicate for viability determinations of A. vinelandii and soil pesticide tracing.
Soil Sampling
Soil was collected from an agricultural plot managed with conventional farming practices (fertilizer, pesticide and tillage use). Soil physical and chemical properties were determined as previously reported (Martínez-Aguilar et al., 2020). The soil used corresponded to sandy loam soil, with the following properties: pH 6.46; organic matter 1.94%; cation exchange capacity 13.97 meq/100 g.
Detection of CP in Soil
Pesticide extraction in soil was performed using the QuEChERS (Quick, Easy, Cheap, Effective, Rugged, and Safe) methodology (Lehotay et al., 2007; Association of Official Analytical Chemists, 2013). Samples of 5 g of soil were placed in a 50 mL centrifuge tube, then 10 mL of deionized water was added and allowed to stand for 30 min. Standard solutions of CP 99.5% N-11459 (Chem Service, United States) and its degradation intermediates TCP 99.5% (33972-BCBZ8746) (Sigma Aldrich, USA) and DETP (Sigma Aldrich, United States) were used for pesticide identification and quantification. Samples were automatically injected through an Acquity® Sample-ManagerFTN system into an Acquity® H-Series ultra-high-performance liquid chromatography (UPLC) system (Waters Corporation, United States) equipped with an Acquity® UPLC BEH Phenyl 1.7 µm, 2.1 × 100 mm column, in a volume of 5.0 µL (Waters Corporation, United States). For chromatographic conditions, mobile phase A was 5 mM pH 3.0 ammonium formate, and mobile phase B was 5 mM acetonitrile +0.1% formic acid at a constant flow rate of 0.30 mL min−1, with the following gradient: starting with 90% solvent A and 10% solvent B, reaching 90% solvent B at 5.0 min remaining 10 s and returning to its first constitution at 8 min and remaining for 1 min. The total running time was 9 min. The injection needle of the autosampler was rinsed with a mobile phase after each injection. Nitrogen was used as the desolvation gas at a flow rate of 1000 L h−1. The desolvation temperature was 600°C, and the source temperature was 150°C. Helium was used as collision gas at a flow rate of 1.0 μL min−1. Identification and quantification were performed using ESI + (CP) and ESI - (TCP) probes on a Xevo TQ-S Mass Spectrometer (Waters Corporation, USA) and workstation with MassLynx ™ 4.1 software (Waters Corporation, USA). Ions were monitored using multiple reaction monitoring (MRM) (Conde-Avila et al., 2021).
The results of the verification of the quality and performance of the method used for the determination of CP and its metabolites were satisfactory according to the validated official method of the AOAC (2013). Details on conditions, extraction method quality, and performance parameters are available as Supplementary Material.
Kinetic Analysis
CP degradation was calculated according to first-order reaction kinetics (Zaranyika et al., 2020) given by the following equation:
Where C0 is the initial CP concentration used, Ct the CP concentration at time interval t, k is the rate constant (d−1), and t is the reaction time (d), in this case 14 d.
The half-life or DT50 (t1/2) of CP was estimated by the following equation: t1/2 = ln 2/k. The percentage of degradation was obtained by calculating the reduction in the concentration of CP, considering 250 mg/kg as 100%.
Genotoxicity and Cytotoxicity Bioassay on A. sativum
For the toxicity test, organic A. sativum bulbs with homogeneous sizes were placed in the soil of the different treatments for day one of the experiment until they roots emerged (10 per experimental unit). Once the roots had developed, they were quantified, measured, and used to obtain meristematic cells. The cut apices were processed and stained with orcein for observation under an optical microscope to assess cytotoxicity and genotoxicity (Gallego and Olivero-Verbel, 2021) (Figure 1). Cytotoxicity was estimated based on the mitotic index (MI) values of 100 cells exposed to the soil of each treatments. The MI was expressed as the number of dividing cells per total cells, according to the following equation:
MI = (Total number of dividing cells/Total number of cells examined) × 100.
For the determination of genotoxicity, the presence of micronuclei (MN) and abnormalities such as binucleated cells (BC), elongated nuclei (EN), caryolysis (CAR), and total abnormalities were considered according to the following equation:
Frequency of abnormalities = Total number of abnormal cells/Total number of normal cells.
Percentage Survival of E. foetida and Viability of A. vinelandii in Soil
E. foetida individuals were provided by the Centro de Agroecología (Instituto de Ciencias, BUAP, Puebla, Mexico). They were cultured at 20°C ± 1°C in organic compost and fed with organic vegetables. Adults with a well-developed clitellum (720 g wet mass) were selected and acclimatized in the same substrate used in the experiments. Thirty ±2 adults per replicate were used in the experiments. At the end of the experiment, the surviving worms were collected and cleaned with distilled water for quantification. To estimate the percentage survival of E. foetida, the number of live organisms at 7 and 14 d was quantified considering the total number of adults introduced in the soil treatments.
The viability of A. vinelandii in soil was determined by a plate count performed on 1 g of soil sample for each treatment, in terms of colony forming units (CFU)/g soil. Subsequently, cultures were made with the recovered bacteria for identification (Conde-Avila, 2022).
Statistical Analysis
The normality and homogeneity of variance of the data obtained from continuous quantitative variables were examined using the Anderson-Darling and Levene tests, respectively. Oneway ANOVA followed by Tukey’s multiple comparisons test were applied for response variables (except survival and degradation percentages). A significance level (alpha) of <0.05 was considered for all tests. The data are presented as mean values and standard deviations. For the determination of damage, the total number of abnormalities was analyzed against the control using the chi2 test (c2) and an alpha <0.01. Finally, the effect of CP in the treatments on the abnormalities was analyzed by applying a Pearson correlation test and a Principal Component Analysis (PCA) with the cytotoxicity and genotoxicity variables. The analyses were carried out using Prism 10 GraphPad Software LLC.
Results
Detection of CP in Soil Inoculated With A. vinelandii
The concentrations of CP and its primary intermediate, TCP, were traced in soil samples 14 days after inoculation (dai) with free and encapsulated A. vinelandii. In the control treatment without pesticide, trace amounts of CP were found in the sampled soil (1.12 mg/kg), which were considered for the calculations of the rest of the treatments (Table 1). In the inoculated treatments and the control + CP, a decrease in the initial concentration added (250 mg/kg) was observed. In the control soil + CP, the results showed a slight elimination of CP by natural attenuation. Conversely, inoculation with A. vinelandii in the soil significantly decreased the initial CP concentration in both liquid and encapsulated inoculum treatments compared to the control + CP treatment (Figure 2; Table 1). Notably, when bacteria were inoculated in free form, it resulted in a shorter DT50 (6.8 d) compared to the encapsulated inoculum (8.5 d), both of which were significantly lower compared to the control treatment without inoculum (DT50 13 d). Thus, the inoculum of A. vinelandii accelerated the degradation of CP in soil regardless of the formulation used.

Table 1. Concentration and degradation measurements of chlorpyrifos (CP) in soil 14 days after inoculation (dai) with free and encapsulated A. vinelandii.
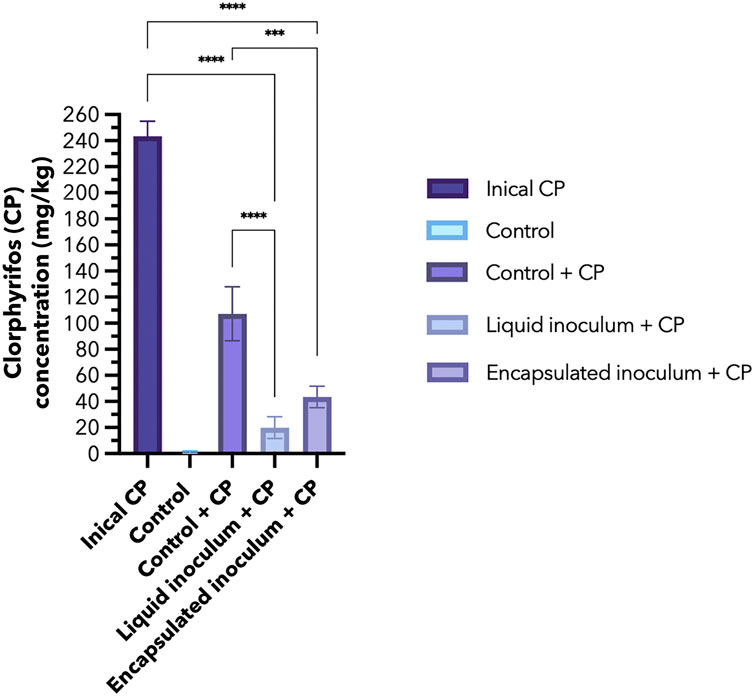
Figure 2. Concentration of chlorpyrifos (CP) in the soil at the beginning of the experiment (initial CP concentration of 250 mg/kg of soil) and its monitoring 14 days after inoculation (dai) in the control treatments (with and without CP) and inoculated with A. vinelandii in liquid and encapsulated formulation.
Regarding the intermediate metabolite TCP, equal quantities were detected in the treatments containing CP, and a significant difference was observed only when compared to the control without CP. However, no accumulation of the intermediate metabolite was observed in proportion to the more substantial degradation of CP in the inoculated treatments. The calculated degradation percentage was 58% by natural attenuation and 92% or 83% after 14 d using the free and encapsulated inoculation with A. vinelandii, respectively.
Viability of A. vinelandii in the Soil
To determine the effectiveness of the inoculation method and the effects of CP, viability counts of A. vinelandii in soil samples were performed (Table 2). Initially, at 7 dai, A. vinelandii colonies were successfully recovered by plate count from both pesticide and nonpesticide treatments using both inoculation methods. However, the viability was higher when using the free-form inoculation (1.3–1.7 × 107 CFU/mL) compared to the encapsulated form application (5.1–5.8 × 106 CFU/mL). Subsequently, at 14 dai, the viability decreased by an order of magnitude in the free-form inoculated treatments (1–1.8 × 106 CFU/mL). This was lower compared to the encapsulated form inoculation (3.2–3.5 × 106 CFU/mL). In both conditions and sampling times, no statistical differences were observed in the treatments with CP compared to the inoculated controls without CP, with the variation primarily attributed to the inoculation method. No A. vinelandii colonies were identified in the treatments without inoculum.

Table 2. Colony-forming units (CFU/mL) obtained from soil samples with CP inoculated with A. vinelandii in liquid and encapsulated form at 7 and 14 days after inoculation (dai).
Toxicity of CP on E. foetida
The adults of E. foetida exposed to the soil of the control treatments without CP, both inoculated and without inoculum, did not show significant differences in the viability of the organisms at 7 and 14 dai of the experiment, maintaining levels above 95% survival. In contrast, the treatments with CP had a dramatic impact on the viability of E. foetida (Figure 3). In the control + CP treatment, within the initial 7 d, the E. foetida population decreased by 87.5%, and by 14 d none of organisms survived exposure to CP. In the treatments inoculated with A vinelandii, survival significantly improved compared to the control + CP. When free A. vinelandii was inoculated into CP-contaminated soils, survival rates were 74% and 34% at 7 and 14 d, respectively, while with encapsulated inoculum, survival rates were 50% and 13% at 7 and 14 dai, respectively.
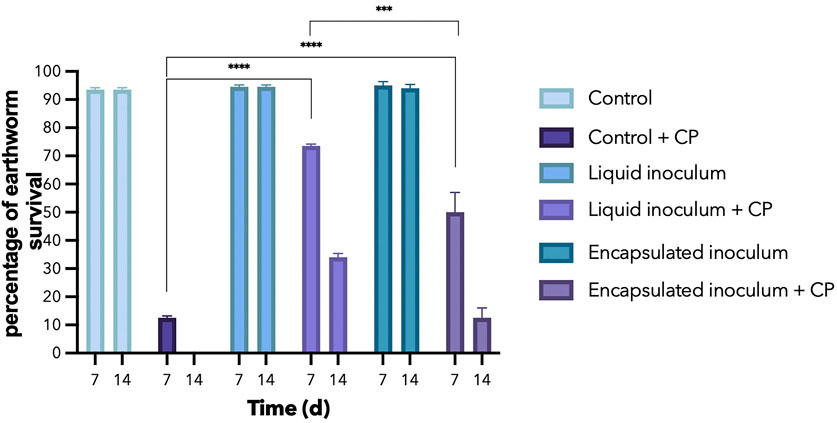
Figure 3. Percentage survival of E. foetida at 7 and 14 days after inoculation (dai) in soil with and without CP and inoculated with A. vinelandii in free and encapsulated formulation.
Cytotoxicity and Genotoxicity on A. sativum
The root length and root number following the exposure of A. sativum to soil with CP and treated with A. vinelandii are presented in Table 3. There were no significant differences in the root length across any of the treatments. In contrast, the number of roots was significantly higher in the control soil with CP (20.66 ± 2.50), followed by the treatment with CP and inoculated with the bacteria in encapsulated formulation (10.66 ± 1.52).

Table 3. Number and length of roots of A. sativum exposed to soil with and without CP and inoculated with A. vinelandii.
The effects of CP and inoculation with free and encapsulated A. vinelandii on the MI in A. sativum root meristematic cells after 14 d of treatment are summarized in Table 4. When counting the number of dividing cells, an increase was found in the positive control with CP, followed by the treatment with CP inoculated with A. vinelandii in encapsulated formulation. However, significant differences were observed in the rest of the treatments compared to the control. Similarly, treatments without pesticide and the one inoculated with A. vinelandii in liquid formulation + CP showed an MI statistically similar to the negative control without CP (7.6 ± 0.45). The highest MI values were obtained in the control + CP treatment (9.33 ± 0.41), followed by the treatment with encapsulated A. vinelandii (8.67 ± 0.35). Thus, MI was increased in treatments in which higher concentrations of CP were detected in the chromatographic analysis (Table 1).
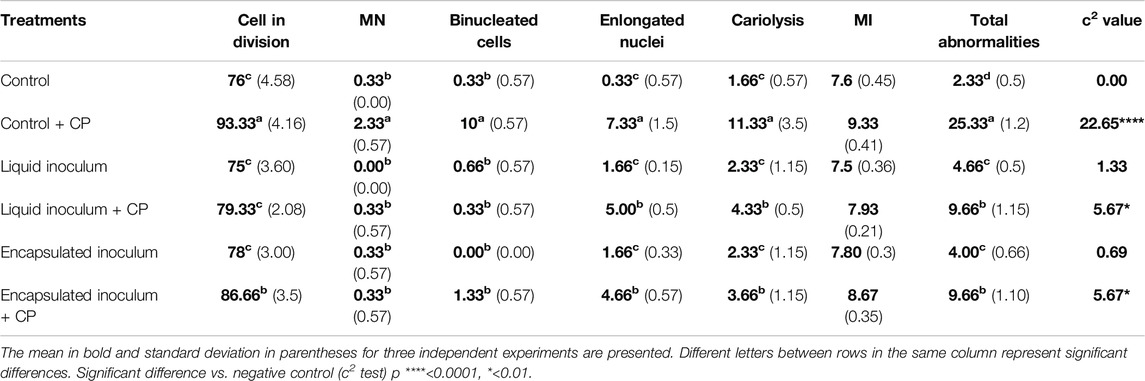
Table 4. Variables of cytotoxic and genotoxic damage observed in A. sativum meristematic cells exposed to soil with CP and treated with inoculums of A. vinelandii.
Finally, to determine the genotoxic damage caused by CP and the effect of A. vinelandii as a treatment for its degradation, the abnormality assay of A. sativum is presented in Table 4. Four types of aberrations, i.e., MN, BC, EN, and CAR, were observed in the dividing meristematic cells. Regarding MN and BC, differences were only observed in the control treatment with CP, where the concentration of CP, according to chromatographic analysis, remained above 100 mg/kg even after 14 d. Similarly, EN and CAR were higher in the control treatment with CP but decreased in the treatments inoculated with A. vinelandii, in liquid and encapsulated formulations. In summary, the number of abnormalities was significantly higher in the control + CP treatment (25.33 ± 1.2) than in the rest of the treatments. It significantly decreased in the treatments inoculated with A. vinelandii (9.66 ± 1.1). The treatments without CP showed a lower total number of abnormalities compared to the control + CP. The total abnormalities correspond in a dependent manner to the concentration of CP detected in each treatment at 14 d (Figure 2; Table 1).
Additionally, to determine the level of correspondence between CP concentration and its toxic effects in A. sativum, a positive correlation (r2 = 0.95, p = 0.02) was found between total abnormalities and CP concentration in the soil of the treatments (Figure 4A). Finally, the analysis of c2 confirmed the genotoxic effects caused by CP and a significant decrease of damage when A. vinelandii inocula were used, in contrast to the Control + CP treatment where only native microbiota were present. When analyzing the different toxicity variables using PCA, two factors explained 92.43% of the variance. We identified as the main factor the variables of CP concentration and abnormalities that explained 79.46% of the total variability (Figure 4B). As the second factor, the variables of root development represented 12.43% of the variance. The above indicates a more significant effect of genotoxicity on cytotoxicity that decreases as a function of CP concentration in the different treatments, especially those inoculated with A. vinelandii, since in the soil with native microbiota (control + CP), the damage persisted despite the compound’s degradation (Table 1; Figure 4A).
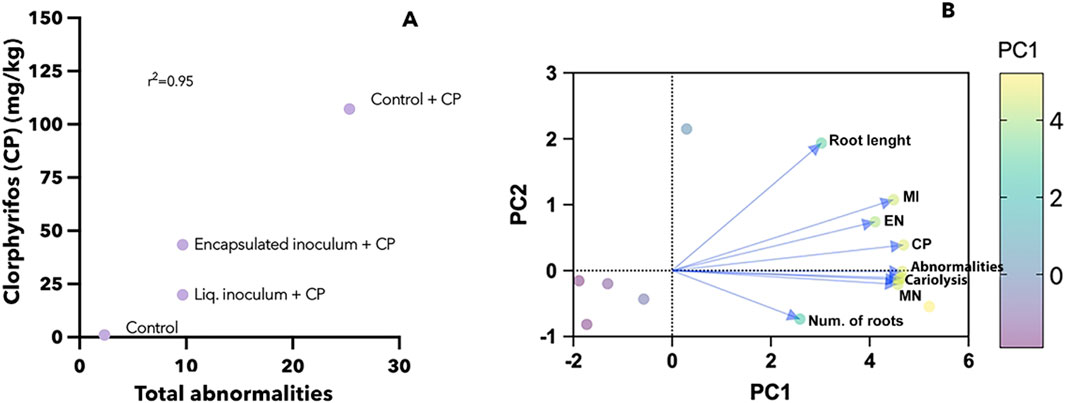
Figure 4. (A) Correlation graph between the concentration of CP in the inoculated treatments and the total abnormalities in cells of A. sativum. (B) Biplot graph of the Principal Component Analysis of the toxicity variables of the treatments. CP, chlorpyrifos; MI, mitotic index; EN, elongated nuclei; MN, micronuclei, abnormalities, cariolysis, root lenght, number of roots.
Discussion
CP Degradation in Soil Inoculated With A. vinelandii
In this study, we show for the first time the degradation of CP in soil inoculated with free and encapsulated A. vinelandii. Previously, it has been documented that some Azotobacter strains have been able to degrade CP in vitro. Chennappa, Sreenivasa, and Nagaraja (2018) showed evidence that Azotobacter tropicalis and Azotobacter salinestris effectively degraded CP in the range of 85%–97.6%, whereas in our work the most effective treatment eliminated 92% in 14 days, but in soil conditions.
In a previous study, we reported that the same strain used in this work, A. vinelandii ATCC 12837, exhibited in vitro degradation of 99.6% of a commercial CP formulation (500 mg/L) with a DT50 of 6 h without affecting its respiration rate, growth, or the accumulation of intermediary metabolites such as TCP, DEPT, or CP oxon (Conde-Avila et al., 2021). In our current study, when inoculated into the soil with initial concentrations of 250 mg/kg, the percentage of degradation was 92.04% and 82.63% using free and encapsulated inoculation with a DT50 of 6.8 and 8.6 d, respectively (Table 1). Importantly, no accumulation of intermediary metabolites was detected, only presence of TCP. That suggests the participation of degradation and mineralization pathways previously described, where CP is hydrolyzed to TCP, followed by reductive dechlorination until its mineralization (Conde-Avila et al., 2021; Bose et al., 2021; Huang et al., 2021). This is significant as it demonstrates the potential of A. vinelandii to tolerate and efficiently degrade the high CP concentrations commonly found in certain agricultural soils.
Adverse affectations on rhizospheric microorganisms involved in N2 fixation are frequently reported when CP is used in soil at doses from 4 to 350 mg/kg (Bhende et al., 2022; Raj and Kumar 2022; Cheng et al., 2023; Amani et al., 2018; Martínez-Toledo, Salmeron, and Gonzalez-Lopez, 1992). Additionally, a decreased in the abundance of cbbLR and cbbLG genes involved in carbon (C) fixation on Acidobacteria, and other affectations on fungi, Actinomycetes, Pseudomonas, and azotobacterias (Amani et al., 2018). In contrast, in this study, A. vinelandii remained viable in soil after 14 days using both inoculation methods. Notably, liquid application favored CP degradation and bacterial viability at 7 dai. The higher percentage of degradation observed with free inoculation can be attributed to the higher number of free cells or CFU in the soil at this early stage (Jabeen et al., 2015; Malla et al., 2023). In encapsulated inoculation, degradation was slightly slower, but viability was better after 14 dai (Table 2). This is consistent with known characteristic of encapsulation in matrices like sodium alginate, which tends to retain microorganisms and release them gradually (Strobel et al., 2018). This gradual release favors sustained bacterial viability and the prolonged effects on CP degradation.
The high degradation rates observed both in vitro (Conde-Avila et al., 2021) and in soil conditions in this study for A. vinelandii can also be attributed to the near-neutral pH in our experiments (pH 6.46). Neutral pH values favor the activity of enzymes related to aromatic ring cleavage, such as organophosphate hydrolases and oxidative stress response enzymes (Duraisamy, Muthusamy, and Balakrishnan, 2018; Farhan et al., 2021). Similarly, Malla et al. (2023) reported that Bacillus cereus AKAD 3-1 removed 94.52% of CP (80 mg/kg) by transforming it into TCP and O, O-diethyl O-hydrogen phosphorothioate and completely mineralizing them into non-toxic by-products within a pH range of 5-7 employing 6.4–7.4 mL inoculum under in vitro conditions (Malla et al., 2023). This is consistent with the results of this research, where the soil pH was 6.46. However, other soil conditions (acidity, higher percentage of organic matter, changes in temperature, among others), and the use of commercial formulations may have contributed to the reduced degradation rates observed in prior in vitro experiments (Deng et al., 2015; Aasfar et al., 2021).
It should be noted that degradation occurred in the treatment without inoculation in this study (Figure 2). This can be attributed to abiotic conditions and the native microbiota, which may exhibit tolerance and capacity for co-metabolic CP degradation in agricultural soils where organophosphorus pesticides have historically been applied (Abraham and Silambarasan, 2018). This is the case for the soil employed in our study since we identified traces of CP in the sampled agricultural soil (Table 1). It would be interesting to consider a microbiome analysis of soils impacted by CP where there is the possibility of degradation by native microbiota. However, it is usually challenging to adapt them to other environments. Other studies have emphasized that stimulation and adaptation of parameters such as pH and humidity are required to favor the elimination of CP (Huang et al., 2021), as happened in this experiment since it would not have occurred with the same efficiency without intervention. Additionally, in this study, the control treatment + CP did not eliminate the acute toxic effects on the bioindicator organisms because the concentration remained always above 100 mg/kg, and the degradation rate was two times slower and about 50% less effective compared to treatments inoculated with A. vinelandii. Thus, a stimulation treatment and adding a microorganism such as A. vinelandii can act effectively and synergistically, reducing the contaminant and the acute toxic effects not eliminated by the native microbiota. In sum, regarding concentration tolerance, efficiency, and the elimination of toxicology effects, A. vinelandii is an excellent candidate for CP degradation and the reduction of its acute effects, considering sandy soil conditions and a pH close to 7.
Toxicity of CP on E. foetida in Soil Inoculated With A. vinelandii
Ecotoxicological effects of CP on various organisms have been previously reported, including bees, fish, microbiota, and earthworms (Organization for Economic Cooperation and Development, 2004; Ali et al., 2008; Vischetti et al., 2020; Hou et al., 2023). Our results indicate that the impact on earthworms is directly related to the CP concentration in the different treatments (Figure 3). The application of CP induced lethal effects, especially in the control + CP treatment, where none of the organisms survived the exposure. This result can be associated with the dose used in the experiment since it has been reported that pesticide doses from 10 to 100 mg/kg typically cause mortality in earthworms (Wang et al., 2015; Ali et al., 2022). Likewise, Krishnaswamy et al. (2021) observed a similar mortality rate and reported alterations in the gut microbiome of the earthworm species Eudrilus euginae. Acute exposure to 180 mg/kg CP caused mortality of E. euginae earthworms like those from this investigation with E. foetida. In our study, applying 250 mg/kg in soil resulted in concentration-dependent mortality, with 100% mortality in the control + CP treatment. Even after 14 dai, the residual CP concentration remained above 100 mg/kg.
When A. vinelandii inoculum was used in the soil, the survival of E. foetida improved significantly (Figure 3). Particularly, with liquid inoculation, the survival percentages were notably higher at 7 and 14 dai. In the encapsulated inoculation, survival also improved but remained slightly lower than in the free inoculation. The difference in survival rates can be attributed to the contrasting residual CP concentration in the different treatments. It is consistent with the rapid degradation of CP by the inoculated bacteria in both liquid and encapsulated form (DT50 of 6 and 8 dai). On the other hand, although natural degradation occurred in the soil control + CP, the period of exposure and concentration of the pesticide were both twice as high (Table 1). These results are consistent with those reported by Zhou et al. (2007), who demonstrated that CP causes acute toxicity (LC50) at concentrations ranging from 91.87 to 118.5 mg/kg in soil, leading to adverse effects on the growth and reproduction of E. foetida dependent on the concentration and exposure period. Damage to earthworms originating from pesticides such as CP is associated with physiological and developmental abnormalities by acting on the inhibition of enzymes such as AChE and butyrylcholinesterase (BChE) in acute exposure (Arguedas et al., 2018). In addition, both CP and TCP cause severe oxidative stress and irreversible DNA damage in E. foetida (Zhu et al., 2020; Hou et al., 2023).
Survival results may vary between species or due to the conditions or products employed. v.g., Ju et al. (2023) similarly observed a concentration-dependent effect of CP on the growth of Lumbricus terrestris but no significant effect on survival or reproduction, suggesting greater sensitivity of E. foetida over other species. De Bernardi et al. (2022) reported effects on weight, reproductive activity, and behavior of E. foetida in soil with CP. They showed a pesticide dose-dependent damage trend but did not report bioaccumulation effects. However, bioaccumulation could explain the damaging effect even in treatments where the concentration of CP decreased over time. CP has the capacity to bioaccumulate in biota, enhancing the toxicity effect on earthworms and other organisms at non-lethal doses. The value of a substance’s octanol-water partition coefficient (KOW) is related to its adsorption capacity or bioaccumulation potential in biota. A low KOW value (<1) indicates solubility, easy biodegradation, and therefore low bioaccumulation. On the contrary, a high KOW value (>5) indicates higher adsorption capacity in fatty tissues, soil, and sediments. The KOW of CP is 4.7, which indicates that it is a substance with high bioaccumulation capacity; however, it is a little-explored topic that could provide more information on pesticide effects on Eisenia spp. at non-lethal doses (Hites, 2021).
Cytotoxic and Genotoxic Effects on A. sativum
Toxicity tests with Allium spp. are validated by the United Nations Environment Programme (UNEP) and the United States Environmental Protection Agency (USEPA) (Bonciu et al., 2018), and are relevant for extrapolations to animal, human and environmental health (Saxena, Gupta, and Murthy, 2010; Ullah et al., 2018; Pereira et al., 2020; Onuminya and Eze, 2019). Allium cepa and A. sativum are the most used species to test pesticide’s toxicity in vitro (Rosculete et al., 2019; Onuminya and Eze, 2019; Camilo-Cotrim et al., 2022; Ghisi et al., 2023). Despite this, research evaluating soil conditions and the effect of commercial pesticide formulations is scarce (Soodan, Katnoria, and Nagpal, 2012; Datta et al., 2018; Fatma et al., 2018; Mota et al., 2022). The effects caused by organophosphorus pesticides in Allium spp. are usually a reduction of root length and MI (Türkoğlu, 2012; Sinha and Kumar, 2014; Fatma et al., 2018), occurrence of various chromosomal aberrations and genetic damage (Gallego and Olivero-Verbel, 2021; Sheikh et al., 2020).
In this study, CP increased MI in the roots of A. sativum in comparison with the negative control. While a lower MI indicates impairments in plant cell growth by G2 blockade or enzymatic inhibition preventing mitosis (Ciğerci et al., 2015; Mesi and Kopliku, 2015; Gallego and Olivero-Verbel, 2021), an increase in MI is related to an overstimulation of cell division, causing uncontrolled proliferation and even the formation of a more significant number of chromosomal and nuclear abnormalities (Sheikh, Patowary, and Laskar, 2020), as in the results of this research (Table 4; Figures 4A, B). Increased cell division may result from reduced time required for DNA repair (Ghisi et al., 2023) or cytosolic signal transduction, causing cell proliferation and increased abnormalities (Hasegawa, Shimonaka, and Ishihara, 2012; Sheikh, Patowary, and Laskar, 2020). Generally, the genotoxic effect of pesticides usually occurs at concentrations lower than those necessary to produce a cytotoxic effect, similar to what was observed in this research, so it is recommended to accompany both evaluations in ecotoxicity studies (Mesi and Kopliku, 2015). In our case, genotoxicity caused by CP was evident, as dividing meristematic cells showed increased and distinct concentration-dependent abnormalities (Table 4; Figures 4A, B). Abnormalities such as CAR, BC, EN, and MN were identified in the CP treatments, especially in the control + CP, and decreased in the treatments inoculated with A. vinelandii, both in liquid and encapsulated inoculation. The increase in the number of abnormalities and a higher MI than the control due to exposure of A. sativum to CP confirms an accelerated and uncontrolled division where DNA damage cannot be efficiently repaired by cellular mechanisms and results in genetic damage observable through various abnormalities (Ghisi et al., 2023).
DNA damage caused by pesticides has been related to their oxidative effect, primarily resulting from the generation of reactive oxygen species (ROS) within plant cells. These ROS cause lipid peroxidation and damage the DNA chain, thereby obstructing processes like replication, repair, recombination, and transcription. Consequently, it causes various aberrations, including MN formation, membrane deterioration, and, ultimately, cell death (Vischetti et al., 2020; Acar et al., 2022). This has been confirmed by the activation of the plant antioxidant defense system, which manifests as an increases in superoxide dismutase (SOD) and catalase (CAT) enzyme activity in response to the presence of pesticides (Fatma et al., 2018; Kalefetoğlu Macar, 2020; Acar et al., 2022). SOD catalyzes free radical forming (O2) and hydrogen peroxide (H2O2), while CAT contributes to oxidative stress protection of cells by scavenging H2O2 (Acar et al., 2022). The extend of the oxidative response may vary depending on the toxicity or type of pesticides because their stereochemical structures play an important role in recognition and reaction with DNA (Türkoğlu, 2012; Onuminya and Eze, 2019; Acar et al., 2022). Organophosphorus compounds such as CP have two potential electrophilic sites: alkyl and phosphoryl groups. In vitro studies have shown that pesticides have the potential to weakly methylate DNA bases and act as a potent alkylating agent by forming bonds between DNA strands that prevent their replication, thereby destroying actively dividing cells and generating genotoxicity (Ghisi et al., 2023). Moreover, CP can produce clastogenic effects by competing with specific essential ions for enzymes involved in mitosis, favoring the appearance of aberrations and abnormalities (Sinha and Kumar 2014). In our study, CP influenced CAR’s appearance in A. sativum cells with increased CP concentration in soil to a greater extent. This abnormality indicates the dissolution of nucleus due to DNAase activity, which can appear due to various reasons, such as lack of ATP or oxidative stress.
The presence and concentration of CP resulted in a significantly higher number of BC in the treatment groups. Previously, BC have been identified in the presence of pesticides caused by cytokinesis arrest in the cell cycle that can lead to cell death after prolonged exposure (Acar et al., 2022; Ghisi et al., 2023). For EN, this abnormality is usually caused by the inhibition of DNA biosynthesis during the S phase of the mitotic cell cycle (Firbas and Amon, 2014; Olaru et al., 2020), or by the interaction of the pesticide with nucleoproteins involved in spindle elongation, microtubule dynamics, and chromosomal movement. Enzymes related to such biological processes include DNA and RNA polymerase, DNA gyrase, and kinases (Ciğerci et al., 2015).
Finally, the occurrence of MN indicates defects in mitotic segregation or errors in DNA replication that generate acentric chromosome fragments or inactive centromere (Tartar et al., 2006; Fernandes, Mazzeo, and Marin-Morales, 2007) and contribute to genomic instability due to loss of information in cells that continue replication (Sinha and Kumar 2014).
Notably, despite the CP´s cytotoxic and genotoxic effects, in treatments where A. vinelandii was inoculated, MI decreased to a level similar to that of the negative control, and total abnormalities decreased in relation to the lower concentration of CP. This suggests that the rapid degradation of CP in soil treated with A. vinelandii, mitigated the damage caused by CP in A. sativum. In particular, the decrease of EN and CAR compared to the control, as well as the consistent occurrence of MN, BC, and IM resembled the patterns observed in the negative control.
Conclusion
The results of this study provide toxicological data from the evaluation of the use of A. vinelandii in the degradation of commercially formulated CP in agricultural soil. Applying A. vinelandii inoculums as a bioremediation treatment effectively reduced the concentration of CP in soil and its toxic effects. Consequently, adopting of a bioremediation approach with the rhizobacterium A. vinelandii represents a sustainable and effective alternative for the rapid degradation of CP in agricultural soil, reducing acute effects without the formation of toxic metabolites. Toxicological monitoring in soil biota is essential for generating alternatives to pesticide contamination, such as CP. In this context, A. sativum is a relevant and practical bioindicator for in situ soil health monitoring in organic and conventional farming systems. This is important since assessing the impact on soil biota and bioindicators is vital to avoid underestimating the short- and long-term pesticides’ effects. The development and implementation of this and other novel techniques for PGPR inoculation, as well as the evaluation of their effectiveness in the degradation of pesticides in soil is recommended. The results of experiments such as those presented here are essential for food security, as they contribute to the monitoring and understanding the effect of pesticides to improve soil quality and generate alternatives for sustainable agriculture.
Data Availability Statement
The original contributions presented in the study are included in the article/Supplementary Material, further inquiries can be directed to the corresponding author.
Ethics Statement
The manuscript presents research on animals that do not require ethical approval for their study.
Author Contributions
Conceptualization, Investigation, Data curation, Formal analysis, Visualization and Writing - original draft: VC. Methodology, Supervision, Funding acquisition, Resources, Review: CM. Supervision, Writing Review and editing: YN. Investigation, Data curation: PB. All authors read and approved the final manuscript. All authors contributed to the article and approved the submitted version.
Funding
The author(s) declare that no financial support was received for the research, authorship, and/or publication of this article.
Conflict of Interest
The authors declare that the research was conducted in the absence of any commercial or financial relationships that could be construed as a potential conflict of interest.
Generative AI Statement
The author(s) declare that no Generative AI was used in the creation of this manuscript.
Acknowledgments
We are thankful to the Mexican Council for Humanities, Sciences and Technologies (CONAHCyT) for providing a postdoctoral fellowship to VC. We appreciate the support provided by the Academic Staff of Biomedical and Biotechnological Sciences of the Universidad Autónoma de Occidente, as well as the Coordinación General para el Fomento a la Investigación Científica e Innovación del Estado de Sinaloa (CONFIE).
Supplementary Material
The Supplementary Material for this article can be found online at: https://www.frontierspartnerships.org/articles/10.3389/sjss.2025.14033/full#supplementary-material
References
Aasfar, A., Bargaz, A., Yaakoubi, K., Hilali, A., Bennis, I., Zeroual, Y., et al. (2021). Nitrogen-Fixing Azotobacter Species as Potential Soil Biological Enhancers for Crop Nutrition and Yield Stability. Front. Microbiol. 12, 628379. doi:10.3389/fmicb.2021.628379
Abraham, J., and Silambarasan, S. (2016). Biodegradation of Chlorpyrifos and its Hydrolysis Product 3,5,6-Trichloro-2-Pyridinol Using a Novel Bacterium Ochrobactrum Sp. JAS2: A Proposal of its Metabolic Pathway. Pestic. Biochem. Physiol. 126, 13–21. doi:10.1016/j.pestbp.2015.07.001
Abraham, J., and Silambarasan, S. (2018). Biodegradation of Chlorpyrifos and 3,5,6-Trichloro-2-Pyridinol by Fungal Consortium Isolated from Paddy Field Soil. Environ. Eng. Manag. J. 17 (3), 523–528. doi:10.30638/eemj.2018.052
Acar, A., Singh, D., and Srivastava, A. K. (2022). Assessment of the Ameliorative Effect of Curcumin on Pendimethalin-Induced Genetic and Biochemical Toxicity. Sci. Rep. 12 (1), 2195. doi:10.1038/s41598-022-06278-5
Akbar, S., and Sultan, S. (2016). Soil Bacteria Showing a Potential of Chlorpyrifos Degradation and Plant Growth Enhancement. Braz J. Microbiol. 47, 563–570. doi:10.1016/j.bjm.2016.04.009
Ali, D., Nagpure, N. S., Kumar, S., Kumar, R., and Kushwaha, B. (2008). Genotoxicity Assessment of Acute Exposure of Chlorpyrifos to Freshwater Fish Channa punctatus(Bloch) Using Micronucleus Assay and Alkaline Single-Cell Gel Electrophoresis. Chemosphere 71 (10), 1823–1831. doi:10.1016/j.chemosphere.2008.02.007
Ali, N., Zaheen, Z., Baba, Z. A., Ali, S., Rasool, S., Sheikh, T. A., et al. (2022). Assessment of Pesticide Toxicity on Earthworms Using Multiple Biomarkers: A Review. Environ. Chem. Lett. 20, 2318–2340. doi:10.1007/s10311-022-01386-0
Amani, F., Safari, S. A. A., Ebrahimi, F., and Nazarian, S. (2018). The Effect of Chlorpyrifos on Frequency of Some Microorganisms in Soil in the Laboratory Condition. J. Soil Biol. 6 (1), 87–100. doi:10.22092/sbj.2018.117150
Apriliya, I., and Mulyawan, R. (2022). Utilization of Azotobacter as Bio-Fertilizer to Support Sustainable Agriculture. Int. J. Chem. Bio Sci. (IJCBS) 21, 197–206. Available online at: https://repo-dosen.ulm.ac.id/bitstream/handle/123456789/26323/24-IJCBS-22-21-24_(1).pdf?sequence=1 (Accessed October 20, 2024).
Arguedas, R., Gómez, A., Barquero, M. D., Chacón, D., Corrales, G., Hernández, S., et al. (2018). Effect of Exposition to Chlorpyrifos upon Plasmatic Cholinesterases, Hematology and Blood Biochemistry Values in Bothrops asper (Serpentes: Viperidae). Chemosphere 205, 209–214. doi:10.1016/j.chemosphere.2018.04.111
Association of Official Analytical Chemists (AOAC) (2013). Official Method 2007.01 Determination of Pesticide Residues in Foods by Acetonitrile Extraction and Partitioning with Magnesium Sulfate. Available online at: https://academic.oup.com/jaoac/article/90/2/485/5657808 (Accessed November 11, 2024).
Aung, S., Talib, A. N., Abdullah, N. Z., and Zainone, Z. M. (2022). Mechanism of Chlorpyrifos Induced Chronic Nephrotoxicity. IIUM Med. J. Malays. 21 (4). doi:10.31436/imjm.v21i4.2023
Bashan, Y., de-Bashan, L. E., Prabhu, S. R., and Hernandez, J. P. (2014). Advances in Plant Growthpromoting Bacterial Inoculant Technology: Formulations and Practical Perspectives (1998–2013). Plant Soil 378, 1–33. doi:10.1007/s11104-013-1956-x
Bhende, R. S., Jhariya, U., Srivastava, S., Bombaywala, S., Das, S., and Dafale, N. A. (2022). Environmental Distribution, Metabolic Fate, and Degradation Mechanism of Chlorpyrifos: Recent and Future Perspectives. Appl. Biochem. Biotechnol. 194, 2301–2335. doi:10.1007/s12010-021-03713-7
Bolaños-Dircio, A., Segura, D., Toribio-Jiménez, J., Toledo-Hernández, E., Ortuño-Pineda, C., Ortega-Acosta, S. A., et al. (2022). Cysts and Alkylresorcinols of Azotobacter Vinelandii Inhibit the Growth of Phytopathogenic Fungi. Chil J Agric Res 82 (4), 658–662. doi:10.4067/S0718-58392022000400658
Bonciu, E., Firbas, P., Fontanetti, C. S., Wusheng, J., Karaismailoğlu, M. C., Liu, D., et al. (2018). An Evaluation for the Standardization of the Allium cepa Test as Cytotoxicity and Genotoxicity Assay. Caryologia 71 (3), 191–209. doi:10.1080/00087114.2018.1503496
Bose, S., Kumar, P. S., and Vo, D. V. (2021). A Review on the Microbial Degradation of Chlorpyrifos and its Metabolite TCP. Chemosphere 283, 131447. doi:10.1016/j.chemosphere.2021.131447
Camilo-Cotrim, C. F., Bailão, E. F. L. C., Ondei, L. S., Carneiro, F. M., and Almeida, L. M. (2022). What Can the Allium cepa Test Say about Pesticide Safety? A Review. Environ. Sci. Pollut. Res. 29, 48088–48104. doi:10.1007/s11356-022-20695-z
Cheng, C., Liu, W., Hou, K., Zhang, J., Du, Z., Li, B., et al. (2023). Ecological Safety Evaluation of Chlorpyrifos on Agricultural Soil: Effects on Soil Microbes. Appl. Soil Ecol. 189, 104954. doi:10.1016/j.apsoil.2023.104954
Chennappa, G., Sreenivasa, M., and Nagaraja, H. (2018). “Azotobacter salinestris: A Novel Pesticide-Degrading and Prominent Biocontrol PGPR Bacteria,” in Microorganisms for Green Revolution. Microorganisms for Sustainability. Editors D. Panpatte, Y. Jhala, H. Shelat, and R. Vyas (Singapore: Springer), 7, 23–43. doi:10.1007/978-981-10-7146-1_2
Chennappa, G., Udaykumar, N., Vidya, M., Nagaraja, H., Amaresh, Y., and Sreenivasa, M. (2019). Azotobacter—a Natural Resource for Bioremediation of Toxic Pesticides in Soil Ecosystems. New Future Dev. Microb. Biotechnol. Bioeng., 267–279. doi:10.1016/B978-0-444-64191-5.00019-5
Ciğerci, İ. H., Liman, R., Özgül, E., and Konuk, M. (2015). Genotoxicity of Indium Tin Oxide by Allium and Comet Tests. Cytotechnology 67 (1), 157–163. doi:10.1007/s10616-0139673-0
Conde-Avila, V., Ortega-Martínez, L. D., Loera, O., Girgis El Kassis, E., García Dávila, J., Martínez Valenzuela, C., et al. (2020). Pesticides Degradation by Immobilised Microorganisms. Int. J. Enviro Anal. Chem. 101 (15), 2975–3005. doi:10.1080/03067319.2020.1715375
Conde-Avila, V., Ortega-Martínez, L. D., Loera, O., Pérez-Armendáriz, B., and Martínez Valenzuela, C. (2022). Encapsulation of Azotobacter Vinelandii ATCC 12837 in Alginate-Na Beads as a Tomato Seedling Inoculant. Curr. Microbiol. 79, 112. doi:10.1007/s00284-022-02797-6
Conde-Avila, V., Peña, C., Pérez-Armendáriz, B., Loera, O., Martínez Valenzuela, C., Leyva Morales, J. B., et al. (2021). Growth, Respiratory Activity and Chlorpyrifos Biodegradation in Cultures of Azotobacter Vinelandii ATCC 12837. Amb. Express 11, 177. doi:10.1186/s13568-021-01339-w021-01339-w
Datta, S., Singh, J., Singh, J., Singh, S., and Singh, S. (2018). Assessment of Genotoxic Effects of Pesticide and Vermicompost Treated Soil with Allium cepa Test. Sustain Environ. Res. 28 (4), 171–178. doi:10.1016/j.serj.2018.01.005
De Bernardi, A., Marini, E., Casucci, C., Tiano, L., Marcheggiani, F., Ciani, M., et al. (2022). Ecotoxicological Effects of a Synthetic and a Natural Insecticide on Earthworms and Soil Bacterial Community. Environ. Adv. 8, 100225. doi:10.1016/j.envadv.2022.100225
Deng, S., Chen, Y., Wang, D., Shi, T., Wu, X., Ma, X., et al. (2015). Rapid Biodegradation of Organophosphorus Pesticides by Stenotrophomonas Sp. G1. J. Hazard Mater 297, 17–24. doi:10.1016/j.jhazm.at.2015.04.052
Devianto, L. A., Latunussa, C. E. L., Helmy, Q., and Kardena, E. (2020). Biosurfactants Production Using Glucose and Molasses as Carbon Sources by Azotobacter Vinelandii and Soil Washing Application in Hydrocarbon-Contaminated Soil. IOP Conf. Ser. Earth Environ. Sci. 475, 012075. doi:10.1088/1755-1315/475/1/012075
Duraisamy, K., Muthusamy, S., and Balakrishnan, S. (2018). An Eco-Friendly Detoxification of Chlorpyrifos by Bacillus Cereus MCAS02 Native Isolate from Agricultural Soil, Namakkal, Tamil Nadu, India. Biocatal. Agric. Biotechnol. 13, 283–290. doi:10.1016/j.bcab.2018.01.001
Elzakey, E. M., El-Sabbagh, S. M., Eldeen, E. E. S. N., Adss, I. A. A., and Nassar, A. M. K. (2023). Bioremediation of Chlorpyrifos Residues Using Some Indigenous Species of Bacteria and Fungi in Wastewater. Environ. Monit. Assess. 195, 779. doi:10.1007/s10661-023-11341-3
EPA. Environmental Protection Agency (2023). Chlorpyrifos, US Environmental Protection Agency. Available online at: https://www.epa.gov/ingredients-used-pesticide-products/chlorpyrifos (Accessed September 22, 2023).
Farhan, M., Ahmad, M., Kanwal, A., Butt, Z. A., Khan, Q. F., Raza, S. A., et al. (2021). Biodegradation of Chlorpyrifos Using Isolates from Contaminated Agricultural Soil, its Kinetic Studies. Sci. Rep. 11, 10320–10414. doi:10.1038/s41598-021-88264x
Fatma, F., Verma, S., Kamal, A., and Srivastava, A. (2018). Phytotoxicity of Pesticides Mancozeb and Chlorpyrifos: Correlation with the Antioxidative Defence System in Allium cepa. Physiol. Mol. Biol. Plants 24 (1), 115–123. doi:10.1007/s12298-017-0490-3
Fernandes, T. C. C., Mazzeo, D. E. C., and Marin-Morales, M. A. (2007). Mechanism of Micronuclei Formation in Polyploidizated Cells of Allium cepa Exposed to Trifluralin Herbicide. Pestic. Biochem. Phys. 88 (3), 252–259. doi:10.1016/j.pestbp.2006.12.003
Firbas, P., and Amon, T. (2014). Chromosome Damage Studies in the Onion Plant Allium cepa L. Caryologia 67 (1), 25–35. doi:10.1080/00087114.2014.891696
Foong, S. Y., Ma, N. L., Lam, S. S., Peng, W., Low, F., Lee, B. H. K., et al. (2020). A Recent Global Review of Hazardous Chlorpyrifos Pesticide in Fruit and Vegetables: Prevalence, Remediation and Actions Needed. J. Hazard Mater 400, 123006. doi:10.1016/j.jhazmat.2020.123006
Gallego, J. L., and Olivero-Verbel, J. (2021). Cytogenetic Toxicity from Pesticide and Trace Element Mixtures in Soils Used for Conventional and Organic Crops of Allium cepa L. Environ. Pollut. 276, 116558. doi:10.1016/j.envpol.2021.116558
Ghisi, N. C., Silva, V. B., Roque, A. A., and Oliveira, E. C. (2023). Integrative Analysis in Toxicological Assessment of the Insecticide Malathion in Allium cepa L. System. Braz J. Biol. 83, e240118. doi:10.1590/1519-6984.240118
Gomathy, M., Sabarinathan, K. G., Subramanian, K. S., Ananthi, K., Kalaiyarasi, V., Jeyshri, M., et al. (2021). “Rhizosphere: Niche for Microbial Rejuvenation and Biodegradation of Pollutants,” in Microbial Rejuvenation of Polluted Environment. Microorganisms for Sustainability. Editors D. G. Panpatte, and Y. K. Jhala (Singapore: Springer), 25, 1–22. doi:10.1007/978-981-15-7447-4_1
Hasegawa, G., Shimonaka, M., and Ishihara, Y. (2012). Differential Genotoxicity of Chemical Properties and Particle Size of Rare Metal and Metal Oxide Nanoparticles. J. Appl. Toxicol. 32, 72–80. doi:10.1002/jat.1719
Hindersah, R., Subarja, O. V., Suryatmanm, P., Sudirja, R., Karuniawan, A., and Hidayat, Y. (2023). Reducing Pb Accumulation in Roots of Sweet Potato under Low Leadcontaminated Soil by Azotobacter Inoculation. J. Degrad. Min. Land Manage 10 (2), 4271–4280. doi:10.15243/jdmlm.2023.102.4271
Hites, R. A. (2021). The Rise and Fall of Chlorpyrifos in the United States. Environ Sci and Technol 55 (3), 1354–1358. doi:10.1021/acs.est.0c06579
Hou, K., Cheng, C., Shi, B., Liu, W., Du, Z., Li, B., et al. (2023). New Insights into the Effects of Chlorpyrifos on Soil Microbes: Carbon and Nitrogen Cycle Related Microbes in Wheat/maize Rotation Agricultural Field. Environ. Pollut. 318, 120908. doi:10.1016/j.envpol.2022.120908
Huang, Y., Zhang, W., Pang, S., Chen, J., Bhatt, P., Mishra, S., et al. (2021). Insights into the Microbial Degradation and Catalytic Mechanisms of Chlorpyrifos. Environ. Res. 194, 110660. doi:10.1016/j.envres.2020.110660
Jabeen, H., Iqbal, S., Anwar, S., and Parales, R. (2015). Optimization of Profenofos Degradation by a Novel Bacterial Consortium PBAC Using Response Surface Methodology. Int. Biodeterior. Biodegr. 100, 89–97. doi:10.1016/j.ibiod.2015.02.022
Jayaraman, P., Naveen Kumar, T., Maheswaran, P., Sagadevan, E., and Arumugam, P. (2012). In vitro Studies on Biodegradation of Chlorpyrifos by Trichoderma Viride and T. Harzianum. J Pure Appl Microbiol 6, 1465–1474. Available online at: https://microbiologyjournal.org/invitro-studies-on-biodegradation-of-chlorpyrifos-by-trichoderma-viride-and-tharzianum/ (Accessed November 12, 2024).
Ju, H., Yang, X., Osman, R., and Geissen, V. (2023). Effects of Microplastics and Chlorpyrifos on Earthworms (Lumbricus terrestris) and Their Biogenic Transport in Sandy Soil. Environ. Pollut. 316 (1), 120483. doi:10.1016/j.envpol.2022.120483
Kalefetoğlu Macar, T. (2020). Investigation of Cytotoxicity and Genotoxicity of Abamectin Pesticide in Allium cepa L. Environ. Sci. Pollut. Res. 28 (2), 2391–2399. doi:10.1007/s11356-020-10708-0
Khalid, S., Han, J. I., Hashmi, I., Hasnain, G., Ahmed, M. A., Khan, S. J., et al. (2018). Strengthening Calcium Alginate Microspheres Using Polysulfone and its Performance Evaluation: Preparation, Characterization and Application for Enhanced Biodegradation of Chlorpyrifos. Sci. Total Environ. 631-632, 1046–1058. doi:10.1016/j.scitotenv.2018.03.101
Kisvarga, S., Hamar-Farkas, D., Ördögh, M., Horotán, K., Neményi, A., Kovács, D., et al. (2023). The Role of the Plant–Soil Relationship in Agricultural Production— with Particular Regard to PGPB Application and Phytoremediation. Microorganisms 11, 1616. doi:10.3390/microorganisms11061616
Krishnaswamy, V. G., Jaffar, M. F., Sridharan, R., Ganesh, S., Kalidas, S., Palanisamy, V., et al. (2021). Effect of Chlorpyrifos on the Earthworm Eudrilus Euginae and Their Gut Microbiome by Toxicological and Metagenomic Analysis. World J. Microbiol. Biotechnol. 37, 76. doi:10.1007/s11274-021-03040-3
Kumar, A., Sharma, A., Chaudhary, P., and Gangola, S. (2021). Chlorpyrifos Degradation Using Binary Fungal Strains Isolated from Industrial Waste Soil. Biologia 76, 3071–3080. doi:10.1007/s11756-021-00816-8
Lehotay, S. J., O’Neil, M., Tully, J., García, A. V., Contreras, M., Mol, H., et al. (2007). Determination of Pesticide Residues in Foods by Acetonitrile Extraction and Partitioning with Magnesium Sulfate: Collaborative Study. J. AOAC Int. 90 (2), 485–520. doi:10.1093/jaoac/90.2.485
Lewis, R. E. (2022). “Chapter 1 - Plant Growth Promotion by Rhizosphere Dwelling Microbes,” in Rhizosphere Engineering. Editors D. R. Chandra, and P. Kumar (Academic Press), 1–17. doi:10.1016/B978-0-323-89973-4.00012-0
Li, J., Fang, B., Ren, F., Xing, H., Zhao, G., Yin, X., et al. (2020). TCP Structure Intensified the Chlorpyrifos-Induced Decrease in Testosterone Synthesis via LH-LHR- PKA-CREB-Star Pathway. Sci. Total Environ. 726, 138496. doi:10.1016/j.scitotenv.2020.138496
Malla, M. A., Dubey, A., Kumar, A., Yadav, S., and Kumari, S. (2023). Modeling and Optimization of Chlorpyrifos and Glyphosate Biodegradation Using RSM and ANN: Elucidating Their Degradation Pathways by GC-MS Based Metabolomics. Ecotoxi Environ. Saf. 252, 114628. doi:10.1016/j.ecoenv.2023.114628
Mankar, K. M., Sahay, S., and Gothawal, R. (2020). Potential of Azotobacter in Sustainable Agriculture. J. Adv. Sci. Res. 11 (6), 01–09. Available online at: https://sciensage.info/index.php/JASR/article/view/1416 (Accessed September 25, 2024).
Martínez-Aguilar, F. B., Guevara-Hernández, F., Aguilar-Jimenez, C. E., RodríguezLarramendi, L. A., Reyes-Sosa, M. B., and La O-Arias, M. A. (2020). Caracterización físico-química y biológica del suelo cultivado con maíz en sistemas convencional, agroecológico y mixto en la Frailesca, Chiapas. Terra Latinoam. 38, 871–881. doi:10.28940/terra.v38i4.793
Martínez-Toledo, M. V., Salmeron, V., and Gonzalez-Lopez, J. (1992). Effect of the Insecticides Methylpyrimifos and Chlorpyrifos on Soil Microflora in an Agricultural Loam. Plant Soil 147, 25–30. doi:10.1007/BF00009367
Mesi, D., and Kopliku, D. (2015). Cyto- and Genotoxic Activity of Pesticide Cypermex Plus 550 EC on. Allium cepa L. J. Inter Environ. App Sci. 10, 475–481. Available online at: https://www.semanticscholar.org/paper/Cyto-and-Genotoxic-Activity-of-Pesticide-Cypermex-Mesi-Kopliku/8bba3d192f22cc2cf3211aba2b572b996e2548d8 (Accessed September 25, 2024).
Mota, T. F. M., Sampaio, A. R., Vasconcelos, M. W., and de Castilhos Ghisi, N. (2022). Allium cepa Test vs. Insecticides: A Scientometric and Meta-Analytical Review. Environ. Sci. Pollut. Res. 29, 42678–42691. doi:10.1007/s11356-021-15953-5
Mousa, N., Adham, A., Merzah, N., and Jasim, S. (2021). Azotobacter Spp. Bioremediation Chemosate. Asian J. Water Environ. Pollut. 18 (3), 103–107. doi:10.3233/AJW210034
Norén, E., Lindh, C., Rylander, L., Glynn, A., Axelsson, J., Littorin, M., et al. (2020). Concentrations and Temporal Trends in Pesticide Biomarkers in Urine of Swedish Adolescents, 2000–2017. J. Expo. Sci. Environ. Epidemiol. 30, 756–767. doi:10.1038/s41370-020-0212-8
Organization for Economic Cooperation and Development (OECD) (2004). Test No. 222: Earthworm Reproduction Test (Eisenia fetida/Eisenia andrei). Paris: OECD. doi:10.1787/9789264070325-en
Olaru, A. L., Rosculete, E., Bonciu, E., Rosculete, C. A., and Saracm, I. (2020). Evaluation of the Cytogenetic Effects of Quantis Biostimulant in Allium Sativum Cells. Not. Bot. Horti Agrobot. Cluj-Napoca. 48 (2), 681–691. doi:10.15835/nbha48211788
Onuminya, T. O., and Eze, T. E. (2019). Cytogenotoxic Effects of Cypermethrin on Root Growth: Allium Sativum as a Model System. Asia-Pac J. Mol. Biol. Biotechnol. 27 (4), 54–61. doi:10.35118/apjmbb.2019.027.4.06
Pechen, A. M., and Venturino, A. (2021). Aspectos legales del uso de clorpirifos; Ministerio de Ambiente y Desarrollo Sostenible. Programa de Naciones Unidas para el Desarrollo, 213–227. Available online at: https://ri.conicet.gov.ar/handle/11336/168000 (Accessed November 12, 2024).
Pereira, M. L., Monteiro, C. N., Siqueira, C. F. N., Ribeiro, M. S., Lopes, A. P., Sousa, R. M. S., et al. (2020). Evaluation of Effects of Poincianella Bracteosa (Tul.) L.P. Queiroz Leaves in Allium cepa and Mus musculus. Biotech. Histochem 95 (6), 464–473. doi:10.1080/10520295.2020.1719197
Raj, A., and Kumar, A. (2022). Recent Advances in Assessment Methods and Mechanism of Microbe-Mediated Chlorpyrifos Remediation. Environ. Res. 214 (4), 114011. doi:10.1016/j.envres.2022.114011
Revillas, J., Rodelas, B., Pozo, C., Martínez-Toledo, M., and González-López, J. (2000). Production of B-Group Vitamins by Two Azotobacter Strains with Phenolic Compounds as Sole Carbon Source under Diazotrophic and Adiazotrophic Conditions. J. Appl. Microbiol. 89, 486–493. doi:10.1046/j.1365-2672.2000.01139.x
Rosculete, C. A., Bonciu, E., Rosculete, E., and Olaru, L. A. (2019). Determination of the Environmental Pollution Potential of Some Herbicides by the Assessment of Cytotoxic and Genotoxic Effects on Allium cepa. Int. J. Environ. Res. Public Health 16 (1), 75. doi:10.3390/ijerph16010075
Ruiz-Arias, M. A., Medina-Díaz, I. M., Bernal-Hernández, Y. Y., Barrón-Vivanco, B. S., González-Arias, C. A., Romero-Bañuelos, C. A., et al. (2023). The Situation of Chlorpyrifos in Mexico: A Case Study in Environmental Samples and Aquatic Organisms. Environ. Geochem Health 45, 6323–6351. doi:10.1007/s10653-023-01618-4
Santo, D. E., Dusman, E., da Silva Gonzalez, R., Romero, A. L., dos Santos Gonçalves do Nascimento, G. C., de Souza Moura, M. A., et al. (2023). Prospecting Toxicity of Octocrylene in Allium cepa L. and Eisenia fetida Sav. Environ. Sci. Pollut. Res. 30, 8257–8268. doi:10.1007/s11356-022-22795-2
Saxena, P. N., Gupta, S. K., and Murthy, R. C. (2010). Carbofuran Induced Cytogenetic Effects in Root Meristem Cells of Allium cepa and Allium Sativum: A Spectroscopic approach for Chromosome Damage. Pestic. Biochem. Phys. 96 (2):93–100. doi:10.1016/j.pestbp.2009.09.006
Schenk, G., Mateen, I., Ng, T. K., Pedroso, M. M., Miti´c, N., Jafelicci, M., et al. (2016). Organophosphate-Degrading Metallohydrolases: Structure and Function of Potent Catalysts for Applications in Bioremediation. Coord. Chem. Rev. 317, 122–131. doi:10.1016/j.ccr.2016.03.006
Sheikh, N., Patowary, H., and Laskar, R. A. (2020). Screening of Cytotoxic and Genotoxic Potency of Two Pesticides (Malathion and Cypermethrin) on Allium cepa L. Mol. Cell Toxicol. 16, 291–299. doi:10.1007/s13273-020-00077-7
Silambarasan, S., and Abraham, J. (2014). Efficacy of Ganoderma Sp. JAS4 in Bioremediation of Chlorpyrifos and its Hydrolyzing Metabolite TCP from Agricultural Soil. J. Basic Microbiol. 54 (1), 44–55. doi:10.1002/jobm.201200437
Sinha, V. S., and Kumar, N. (2014). Assessment of Mito-Inhibitory and Genotoxic Effects of Two Organophosphate Pesticides in the Root Tip Cells of Allium cepa L. Ann. Plant Sci. 5 (3), 699–703. Available online at: https://www.annalsofplantsciences.com/index.php/aps/article/view/104 (Accessed October 23, 2024).
Soodan, R. K., Katnoria, J. K., and Nagpal, A. (2012). Allium cepa Root Chromosomal Aberration Assay: An Efficient Test System for Evaluating Genotoxicity of Agricultural Soil. Int. J. Sci. Res. 3 (8), 245–250. Available online at: https://api.semanticscholar.org/CorpusID:4644248 (Accessed October 21, 2024).
Strobel, S. A., Allen, K., Roberts, C., Jimenez, D., Scher, H. B., and Jeoh, T. (2018). Industrially Scalable Microencapsulation of Plant Beneficial Bacteria in Dry Cross-Linked Alginate Matrix. Ind. Biotechnol. 14 (3), 138–147. doi:10.1089/ind.2017.0032
Sun, Y., Pei, J., Chen, X., Lin, M., Pan, Y., Zhang, Y., et al. (2023). The Role of the Gut Microbiota in Depressive-Like Behavior Induced by Chlorpyrifos in Mice. Ecotox Environ. Saf. 250, 114470. doi:10.1016/j.ecoenv.2022.114470
Tartar, G., Kaymak, F., Gokalp, , and Muranli, F. D. (2006). Genotoxic Effects of Avenoxan on Allium cepa L. and Allium sativum L. Caryologia 59 (3):241–247. doi:10.1080/00087114.2006.10797921
Türkoğlu, Ş. (2012). Determination of Genotoxic Effects of Chlorfenvinphos and Fenbuconazole in Allium cepa Root Cells by Mitotic Activity, Chromosome Aberration, DNA content, and Comet Assay. Pestic. Biochem. Phys. 103 (3):224–230. doi:10.1016/j.pestbp.2012.06.001
Ullah, S., Zuberi, A., Alagawany, M., Farag, M. R., Dadar, M., Karthik, K., et al. (2018). Cypermethrin Induced Toxicities in Fish and Adverse Health Outcomes: Its Prevention and Control Measure Adaptation. J. Environ. Manage 206 (15), 863–871. doi:10.1016/j.jenvman.2017.11.076
United Nations Environment Programme (UNEP) (2023). Proposal to List Chlorpyrifos in Annex A to the Stockholm Convention on Persistent Organic Pollutants UNEP/POPS/POPRC.17/4. Available online at: https://www.pops.int/TheConvention/POPsReviewCommittee/Meetings/POPRC17/Overview/tabid/8900/Default.aspx (Accessed September 20, 2023).
Vejan, P., Abdullah, R., Khadiran, T., Ismail, S., and Nasrulhaq, B. (2016). Role of Plant Growth Promoting Rhizobacteria in Agricultural Sustainability—A Review. Molecules 21 (5), 573. doi:10.3390/molecules21050573
Vischetti, C., Casucci, C., De Bernardi, A., Monaci, E., Tiano, L., Marcheggiani, F., et al. (2020). Sub-Lethal Effects of Pesticides on the DNA of Soil Organisms as Early Ecotoxicological Biomarkers Pesticides on the DNA of Soil Organisms as Early Ecotoxicological Biomarkers. Front. Microbiol. Sec Plant Pathog. Interact. 11. 1892. doi:10.3389/fmicb.2020.01892
Wang, K., Mu, X., Qi, S., Chai, T., Pang, S., Yang, Y., et al. (2015). Toxicity of a Neonicotinoid Insecticide, Guadipyr, in Earthworm (Eisenia fetida). Ecotoxicol. Environ. Saf. 114, 17–22. doi:10.1016/j.ecoenv.2014.12.037
Wang, Y., Zhu, Y. C., and Li, W. (2020). Comparative Examination on Synergistic Toxicities of Chlorpyrifos, Acephate, or Tetraconazole Mixed with Pyrethroid Insecticides to Honey Bees (Apis mellifera L.). Environ. Sci. Pollut. Res. 27, 6971–6980. doi:10.1007/s11356-019-07214-3
World Health Organization (WHO) (2015). Human Biomonitoring: Facts and Figures. Regional Office for Europe, Copenhagen, Switzerland: World Health Organization. Available online at: https://apps.who.int/iris/handle/10665/164588 (Accessed October 16, 2023).
Zaranyika, M. F., Matimati, E., and Mushonga, P. (2020). Degradation Kinetics of DDT in Tropical Soils: A Proposed Multi-Phase Zero Order Kinetic Model that Takes into Account Evaporation, Hydrolysis, Photolysis, Microbial Degradation and Adsorption by Soil Particulates. Sci. Afr. 9, e00467. doi:10.1016/j.sciaf.2020.e00467
Zhang, L., Feng, L., Jiang, J., Li, P., Chen, X., Zhang, S., et al. (2021). A Highly Sensitive and Visible-Light-Driven Photoelectrochemical Sensor for Chlorpyrifos Detection Using Hollow Co9S8@CdS Heterostructures. Sens. Actuators B Chem. 348, 130719. doi:10.1016/j.snb.2021.130719
Zhou, S.-P., Duan, C.-Q., Fu, H., Chen, Y.-H, Wang, H.-U., Yu, Z.-F., et al. (2007). Toxicity assessment for chlorpyrifos-contaminated soil with three different earthworm test methods. J. Environ. Sci. 19(7) 854–858. doi:10.1016/S1001-0742(07)60142-9
Keywords: encapsulation, rhizobacteria, toxicity, pesticide, biological degradation
Citation: Conde Avila V, Martínez Valenzuela C, Navarro Noya YE and Bastidas Bastidas PJ (2025) Inoculation With Azotobacter vinelandii Enhanced Chlorpyrifos Degradation and Reduced Cytotoxic and Genotoxic Effects in Soil. Span. J. Soil Sci. 15:14033. doi: 10.3389/sjss.2025.14033
Received: 06 November 2024; Accepted: 28 February 2025;
Published: 21 March 2025.
Edited by:
Avelino Núñez-Delgado, University of Santiago de Compostela, SpainCopyright © 2025 Conde Avila, Martínez Valenzuela, Navarro Noya and Bastidas Bastidas. This is an open-access article distributed under the terms of the Creative Commons Attribution License (CC BY). The use, distribution or reproduction in other forums is permitted, provided the original author(s) and the copyright owner(s) are credited and that the original publication in this journal is cited, in accordance with accepted academic practice. No use, distribution or reproduction is permitted which does not comply with these terms.
*Correspondence: C. Martínez Valenzuela, Y2FtYXZhOUBnbWFpbC5jb20=
†ORCID: V. Conde Avila, orcid.org/0000-0003-2700-8603; C. Martínez Valenzuela, orcid.org/0000-0003-1784-9986; Y. E. Navarro Noya, orcid.org/0000-0002-7191-7815; P. J. Bastidas Bastidas, orcid.org/0000-0003-0419-0481