- Department of Medicine, Laboratory of Translational Immunology, Division of Immunology and Allergy, Geneva University Hospitals and Faculty of Medicine, Geneva, Switzerland
Xenotransplantation represents a potential solution to the shortage of organs for transplantation. The recent advancements in porcine genetic modification have addressed hyperacute and acute vascular rejection; however, challenges persist with regard to delayed xenograft rejection. Porcine endothelial cells (pECs) represent a crucial target in the context of xenograft rejection, which is mediated by cytotoxic lymphocytes. It is crucial to comprehend the manner in which human natural killer (NK) cells and cytotoxic CD8+ T lymphocytes (CTL) recognize and target pECs in order to develop efficacious prophylactic strategies against rejection. The objective of the present review is to synthesize the existing knowledge regarding the mechanisms and techniques employed to modulate xenogeneic responses mediated by human NK cells and CTL. We will elucidate recent methodological advancements, debate potential novel strategies, and emphasize the imperative necessity for further research and innovative approaches to enhance graft survival.
Introduction
Numerous hurdles in xenotransplantation need to be overcome to achieve tolerance of the recipient’s immune system [1–3]. Hyperacute rejection seems to be resolved due to the availability of knockouts of the major porcine xenoantigens such as αGal [4–6]. Even so, acute vascular and delayed xenograft rejection mediated by both the innate and adaptive immune system remain an obstacle to successful xenotransplantation despite novel systemic immunosuppressive regimens.
The endothelium of a vascularized porcine xenograft represents the initial point of contact with the recipient’s blood, which contains a multitude of immune cell subsets. Consequently, a significant objective of contemporary research initiatives is to evaluate the impact of diverse genetic modifications in porcine endothelial cells (pECs) on human immune cells. It was shown that the complement- and coagulation-mediated damage of pECs can be prevented by knocking out the genes of the three major xenocarbohydrates (αGal, Neu5Gc, and Sda), GGTA1, CMAH, and B4GALNT2, respectively [4], by introducing human complement regulators (hCD46, hDC55, and hCD59) [7, 8], and thrombomodulin (hTBM). Overcoming these hurdles of hyperacute and acute vascular rejection, consistent life-supporting function of xenografted hearts for up to 195 days was achieved in a pig-to-non-human primate (NHP) preclinical model with a combination of αGal knockout (GTKO), and transgenic expression of hCD46 and hTBM [9]. Consistent long-term survival was also demonstrated in pig-to-NHP kidney xenotransplantation using 10GE (genetically engineered) source pigs and FDA-approved immunosuppression [10]. These data provide critical supporting evidence for the safety and feasibility of clinical kidney xenotransplantation, even without CD40/CD154 costimulatory blockade. However, it was not further explored in these models, whether responses mediated by natural killer (NK) cells and cytotoxic CD8+ T lymphocytes (CTL) were sufficiently controlled. Further, pig-to-primate experiments are considered as the best-possible pre-clinical constellation for evaluating xenotransplantation, but it is largely unclear to which extent genetic modifications that have been designed for humans remain purposeful in baboons.
The significance of NK cells in xenograft rejection was first shown in the early 90s by Inverardi et al using an ex vivo human-to-rat perfusion model [11]. Subsequently, our research group and others have conducted comprehensive, predominantly in vitro investigations into the function of NK cells in pig-to-human xenotransplantation [12–14]. A comprehensive review of the immunological barriers, including strategies of inhibition, was published by Sykes and Sachs in 2022 emphasizing approaches to promote and induce T cell tolerance in xenograft recipients [2]. The goal of the present review is to focus on our current understanding of the mechanisms involved in xenogeneic responses mediated by human cytotoxic lymphocytes (NK cells and CTL), in the light of recent advances in the field of xenotransplantation, and to discuss potential strategies for prevention of xenograft rejection.
Recruitment and Activation of Cytotoxic Lymphocytes by Porcine Endothelial Cells
Antibody binding, complement deposition, and coagulation proteins activate porcine endothelium leading to the release of pro-inflammatory cytokines such as TNF and IL-6. These cytokines subsequently recruit immune cells to the graft [15, 16]. Activated pECs express E- (CD62E) and P- (CD62P) selectin which bind to L-selectin (CD62L) on human lymphocytes, thereby initiating the rolling phase. Human β2 integrins, such as LFA-1 (CD11a/CD18), interact with immunoglobulin superfamily members (i.e., porcine ICAM-1) enabling firm adhesion of the lymphocytes [17]. It is of particular significance that the human β1 integrin very late antigen-4 (VLA-4; CD49d/CD29) has the capacity to bind to VCAM-1 (CD106) on pECs [17–19], thereby facilitating NK cell and CTL adhesion. Lastly, it has been demonstrated using transwell assays in vitro that transendothelial migration of human leukocytes is dependent on CD99 and the β2 integrin CD18 [18]. Conversely, homotypic interactions mediated through CD31 (PECAM-1) have been shown to be incompatible in the pig-to-human context [19].
NK cells and CTL mediate anti-pig cytotoxicity through lytic granule release (perforin and granzyme B) or the Fas/FasL and TRAIL pathways, causing apoptotic and/or necrotic death of the target cells [20–25]. While sharing the killing mechanisms, the robust response of NK cells towards target cells can in turn initiate and amplify CTL-mediated cytotoxicity [26, 27]. Both produce cytokines like interferon-gamma (IFNγ) and tumour necrosis factor (TNF) [28]. In the recent in vivo studies of pig-to-NHP and pig-to-human (decedent recipients) solid organ transplantation, NK cells were found to be the early producers of IFNγ [29, 30]. An overview of the classical techniques used to evaluate NK cell and CTL xenoresponses in vitro and ex vivo, as well as an overview of the current cutting-edge -omics techniques, such as single-cell RNA sequencing (scRNA-seq) and longitudinal RNA-seq analyses, is presented in Box 1. To assess cell-cell interactions, i.e., lymphocytes adhesion to pECs and transendothelial migration under shear stress, our group has recently established a 3D microfluidic system [31], [Tran T et al., in press].
Box 1 Methods to analyse NK and CTL porcine xenoresponses.
In vitro (for NK and CTL unless said otherwise)
Cytotoxicity release assay. Here, effector cytotoxic cells are co-cultured with porcine target cells (either pPBMC or pEC) previously loaded with 51[Cr] or BADTA which after cellular membrane destruction are released to the culture media and quantified.
CD107a degranulation assay. In this flow cytometry-based assay, the degranulation of lytic granule contents by cytotoxic cells upon encounter with porcine target cells is detected by the surface expression of CD107a thanks to the fusion of lytic granule to the cellular membrane. This assay could be coupled to intracellular cytokine detection.
Adhesion assays using fluorescence time-lapse microscopy. Porcine endothelial cell destruction is quantified in real-time upon encounter with cytotoxic cells. This method allows in addition to study cytotoxic cell displacement on a carpet of pEC and type of cell elimination. The next level is achieved by using a 3D microvessel system covered by a monolayer of pEC where cytotoxic cells first adhere to encounter and destroy their targets under flow.
Mix lymphocyte reaction (MLR). This assay is specific for T cells and measures T-cell responses from the recipient (human or NHP) to xenoantigens. It involves co-culturing responder lymphocytes (recipient) with irradiated or Mitomycin C-treated stimulator cells (donor PBMC) to assess proliferation through thymidine incorporation, CFSE or BrdU staining, or cytokine production by ELISpot or ELISA. Xeno-specific T cells proliferate upon encountering antigens on stimulator cells to which they are specifically reactive, such as SLA-1.
Transendothelial migration (TEM) assay. Is used to study the movement of lymphocytes across the endothelial layer. Endothelial cells are cultured in Transwell insert which has a porous bottom that allows immune cells to migrate through it. Lymphocytes migrate into wells containing a chemoattractant or medium and can be quantified once the transmigration is completed.
Antibody-dependent cell cytotoxicity (ADCC). This assay measures the activation of NK cells via the CD16 receptor, leading to target cell lysis when IgG xenoantibodies present in the recipient’s sera bind to donor-derived cells. It is performed similarly to the cytotoxicity release assay, where donor cells are labeled beforehand.
Ex vivo
Biopsies. Analysis by histology, immunohistochemistry, or immunofluorescence is performed in some cases both during graft acceptance and rejection. This examination evaluates cellular infiltration, tissue architecture, and marker expression to assess immune responses, inflammation, and graft viability. These analyses offer insights into the mechanisms of graft acceptance or rejection, but they are purely descriptive and do not evaluate functional outcomes.
Analysis of CTL and NK cells functions in blood. Here the same assays of cytotoxic release assay, CD107a degranulation, MLR, ADCC.
Omics era
Bulk and single-cell RNA sequencing. As a starting material, either biopsies of a xenograft or recipient’s PBMC are used to measure gene expression across the entire cell population (bulk RNAseq) or for each individual cell within the population (scRNAseq). This allows to identify genes of interest which could be targeted in the xenotransplantation setting.
Spatial transcriptomics of single nucleus/cells. Combines histology and RNAseq. It enables the mapping of gene expression across different regions of a tissue sample, maintaining spatial context. The method can map the immune response at the site of the xenograft. This includes identifying immune cell infiltration patterns and understanding local cytokine and chemokine expression.
Natural Killer Cells
In the context of pig-to-human xenotransplantation, incompatibilities in species-specific molecular interactions can either result in the activation of NK cells or prevent it through a lack of inhibitory signals (Figure 1). NK cells exert their function via two primary mechanisms (i) antibody-dependent cellular cytotoxicity (ADCC) and (ii) direct cytotoxicity. ADCC is initiated when the NK cell receptor CD16 (FcγRIIIA) binds to human antibodies that are directed against xenoantigens expressed on pig endothelium; (ii) in direct cytotoxicity the lack of recognition (“missing self”) of SLA (swine leukocyte antigen) MHC class-I molecules by inhibitory NK cell receptors (CD94/NKG2A, KIRs, and ILT2) [32–34] results in the activation of NK cells. Another immune checkpoint molecule providing inhibitory signals, SIRPα (CD172a), can be expressed on NK cells upon cytokine stimulation [35]. Among different activating NK cell receptors CD2 (LFA-2), NKp44 and NKG2D are involved in both porcine cell lysis and cytokine production [36, 37]. Porcine CD58 may function as an activating ligand for CD2 expressed on human NK cells [38, 39] as evidenced by inhibitory effects of pCD58 blockade on human peripheral blood mononuclear cells (PBMC) cytotoxicity and proliferation in response to porcine cells [40]. The interaction between human NKG2D and porcine ULBP1 (pUBPL1) remains a topic of some debate. The porcine ligand for the activating NK cell receptor NKp44 is still unknown, reported candidates include proliferating cell nuclear antigen (PCNA), heparan sulfate proteoglycans (HSPGs), viral hemagglutinin, MHC class I related chain A (MICA), platelet-derived growth factor (PDGF)-DD, and some HLA-DP molecules [41, 42], however, a widely expressed cellular ligand for NKp44 has remained elusive and controversial. Finally, the impact of glycosylation, in particular sialic acids on the interaction between NK cells and potential target cells needs to be further investigated [43]. The expression of Siglec-7 ligands on target cells can protect them from NK cell-mediated lysis whereas masking of ligands for Siglec-7 and Siglec-9 has been demonstrated to enhance anti-tumour activity of NK cells. This aspect has not yet been evaluated in the context of transplantation.
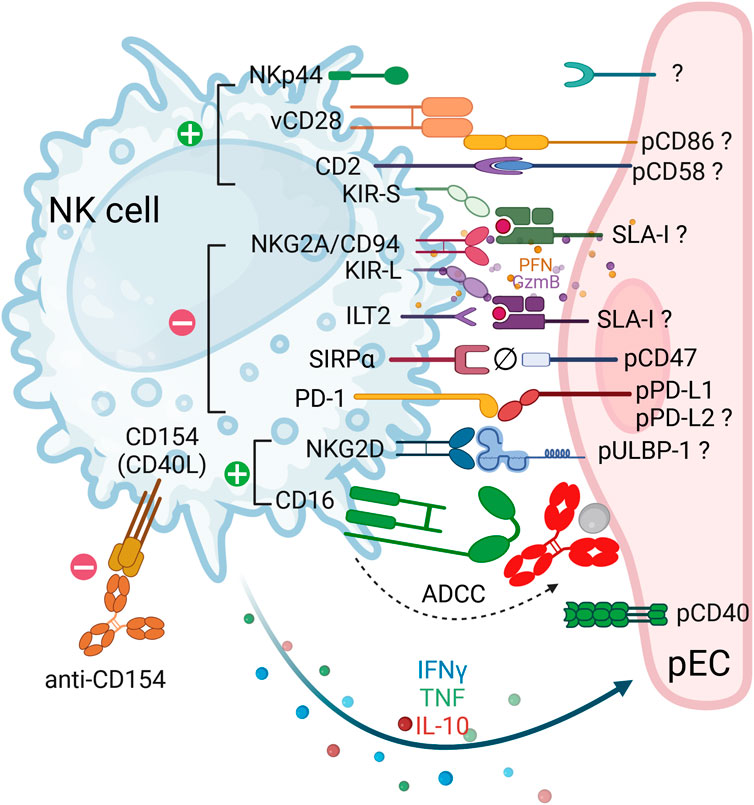
Figure 1. Receptor-ligand interactions between human NK cells and porcine endothelial cells. The cartoon depicts the interactions between human natural killer (NK) cells (blue) and porcine endothelial cells (pEC, pink). In instances where the ligand-receptor pair interaction is functional across species, the corresponding pair is indicated. In cases where the ligand-receptor pair is suspected, or when contradictory data is available, a question mark is added to indicate this. Ligand-receptor interactions that result in either activation or inhibition are represented by green (+) and red (−) circles, respectively. Incompatibility between pCD47 and hSIRPα is symbolized by Ø. The engagement of activating receptors results in the release of lytic granule content (perforins, granzymes and granulysins), which is then followed by the secretion of cytokines (e.g. IFNγ, TNF and IL-10). Co-stimulatory molecules expressed on pEC include CD80/CD86 and PD-L1. NK cells are also capable of expressing these ‘co-stimulatory’ molecules (CD28 and PD-1), thereby regulating their function. Therapeutic strategies that interfere with the costimulatory pathways include anti-CD40L (anti-CD154), as shown in the figure, anti-CD40 and the soluble CTLA-Ig molecule, among others. Finally, NK cells can be strongly activated by the binding of their CD16/FcgRIIIa to antibodies attached to pEC leading to antibody-dependent cellular cytotoxicity (ADCC).
Cytotoxic T Lymphocytes
Unlike NK cells, naïve CD8+ T cells require prior stimulation with porcine antigens target cell elimination [44]. In vitro data show that human T lymphocytes recognize porcine cells via both direct and indirect pathways [45–49]. Direct recognition entails T cell receptor (TCR) interactions with SLA-I/II and co-stimulatory molecules CD80/86 [48–52], via CD2 and CD28 pathways [53] on pECs (Figure 2), whereas indirect recognition involves the presentation of pig antigens by recipient’s antigen-presenting cells (APC) [54]. Moreover, in vitro studies have demonstrated that the proliferative responses of human T cells are enhanced when both porcine cells and human autologous APC are co-cultured, thereby combining direct and indirect recognition mechanisms [55, 56]. Additionally, indirect presentation was described by the recognition of SLA-I within endothelial cell derived extracellular vesicles [52]. CTL not only recognize SLA-I allelic polymorphisms, but they also require the presence of porcine peptides bound to SLA-I for an efficient xenospecific reaction [57–59]. Consequently, specific T cell clones proliferate and differentiate into effector and then memory cells that react rapidly upon the antigen re-exposure [60]. Co-stimulation can also be provided via pCD86 expressed on porcine cells binding CD28 on both CTL and NK cells [61–64], as well as binding of CD154 (CD40L) and CD40 [65, 66] (Figures 1, 2). It is noteworthy that in vitro studies utilizing human and baboon PBMC demonstrated a reduction in T cell responses to GGAT1 KO pig-derived pEC in comparison to wild-type pEC, irrespective of whether the pEC were activated. CD4 and CD8 lymphocytes showed reduced proliferation, cytokine production, and granzyme/perforin content. Nevertheless, the specific mechanisms by which αGal modulates the T cell response remain to be elucidated [67].
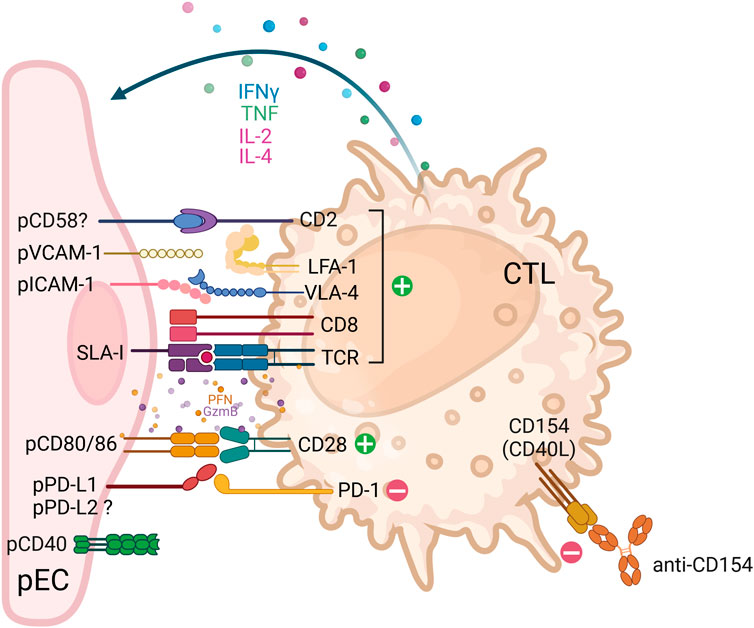
Figure 2. Receptor-ligand interactions between human cytotoxic T lymphocytes (CTL) and porcine endothelial cells. The cartoon depicts the interactions between cytotoxic T lymphocytes (CTL) (CD8+ T cells, orange) with porcine endothelial cells (pEC, pink). In instances where the ligand-receptor pair interaction is functional across species, the corresponding pair is indicated. In cases where the ligand-receptor pair is suspected, or when contradictory data is available, a question mark is added to indicate this. Ligand-receptor interactions that result in either activation or inhibition are represented by green (+) and red (-) circles, respectively. The engagement of activating receptors results in the release of lytic granule content (perforins, granzymes and granulysins), which is then followed by the secretion of cytokines (e.g. IFNγ, TNF, IL-2 and IL-4). The term ‘costimulatory pathways’ was first employed in the context of T cell immunology following TCR engagement (signal 1). The term is used to describe the molecules that are required to further activate or inhibit T cells (signal 2). Such molecules include CD80/CD86 and PD-L1. It is noteworthy that the co-stimulatory CD28 is a dual-function molecule on CTL, capable of binding to CD80/CD86 on pEC, thereby providing activation or to CTLA-4 on helper CD4 T cells, thereby triggering an inhibitory signal. Therapeutic strategies that interfere with the costimulatory pathways include anti-CD40L (anti-CD154), as shown in the figure, anti-CD40 and the soluble CTLA-Ig molecule, among others. Abbreviations: ADCC: antibody-dependent cellular cytotoxicity; CD154: also known as CD40L; CD16/FcgRIIIa: Fc gamma Receptor IIIa; CD2: also known as lymphocyte-function antigen-2 (LFA-2); CTL: cytotoxic T lymphocyte; CTLA-4: cytotoxic T-lymphocyte associated protein 4, also known as CD152 molecule; GzmB: granzyme B; IFNγ: interferon-gamma; IL: interleukin; ILT2: immunoglobulin-like transcript 2, also known as leukocyte immunoglobulin like receptor B1 (LILRB1); KIR: killer cell immunoglobulin-like receptor, -L – inhibitory, -S - activating; LFA-1: lymphocyte function-associated antigen 1, also known as CD11a molecule; NK: natural killer; NKG2A: also known as killer cell lectin like receptor C1 (KLRC1); NKG2D: also known as killer-cell lectin-like receptor K1 (KLRK1); NKp44: natural cytotoxicity triggering receptor 2, also known as NCR2 and CD336 molecule; p: porcine; PD-1: programmed cell death 1 also known as CD279 molecule; PD-L1/2: programmed cell death 1/2 ligand, also known as CD274/CD273; PFN: perforin; SIRPα: signal regulatory protein alpha also known as CD172a molecule; SLA-I: swine leukocyte antigen-1; TCR: T-cell receptor; TNF: tumour necrosis factor; ULBP-1: UL16 binding protein 1; vCD28: variant of CD28; VLA-4: very late antigen-4, integrin formed by alpha4/beta4 subunits (CD49d/CD29 molecules).
Findings in the Recent Studies In Vivo
The findings across various animal models demonstrated the complexity and challenges associated with NK and CTL responses in pig-to-primate xenotransplantation. In baboons that received porcine heart transplants expressing either hCD55 or hCD46, without specific T cell depletion, the rejection process occurred rapidly, within 5–7 days, and was characterized by a high prevalence of macrophages and T cells [68, 69]. Similarly, porcine islet transplantation into cynomolgus monkeys and rhesus macaques resulted in infiltration and rejection, which were primarily mediated by T cells, even before macrophage recruitment into the tissues [70, 71]. In the case of porcine cartilage transplanted into cynomolgus monkeys, up to 90% of the infiltrating cells were T cells, with equal amounts of CD4+ and CD8+ T cells, as observed 1 month post-transplantation [72]. Recent studies have demonstrated the involvement of NK cells and a few adaptive CD3+ cells in porcine kidney xenografts transplanted into brain-dead recipients. NK cells and T cells were identified in the glomeruli 54 h post-transplantation, with transcriptome profiling showing increased expression of genes related to IFNγ response, NK cells (HLA-E and FCGR3A/B), and T cell development (CD81) [73]. Longitudinal bulk RNA-seq of kidney xenografts revealed increased IFNγ signaling as early as 12 h post-transplantation, predominantly attributed to infiltrated NK cells [30]. Single-cell RNA-sequencing of recipient’s PBMCs revealed two distinct waves of immune response with upregulation of genes associated with NK and T cells. This correlated with increased IFNγ expression in NK cells at 12 and 48–53 h [30]. Similar trends were observed through scRNA-seq analysis on day 38 in the first pig-to-human cardiac xenograft recipient. Dominant changes in immune cell type composition across the time points were observed for monocytes, both CD4+ and CD8+ T cells, and NK cells, accompanied by the upregulation of genes related to NK and T cell activation, and IFN responses. However, it was difficult to pinpoint any single etiology for the xenograft dysfunction [74]. The latest multi-omics analyses of samples from two deceased human recipients of 10-gene-edited pig hearts have revealed two distinct scenarios regarding the administration of thymoglobulin and the duration of cold ischemic time. One of the decedents exhibited a lack of evidence of immune cell activation, whereas the other decedent displayed an increase in T and NK cells in both circulating PBMC and xenograft [75]. This notable rise in lymphocytes in one of the recipients may be attributed to the longer ischemic time of the pig heart and the T cell depletion protocol resulting in the expansion of memory T cells and rapid xenograft injury.
Strategies of Inhibition
NK Cells
Based on the regulation of NK cell function predicted by the “missing self” theory and the observation that SLA MHC class I molecules do not appear to interact sufficiently with inhibitory human NK cell receptors, human MHC class I molecules were introduced into pig cells to protect against human NK cytotoxicity [12, 13, 76–78] (Table 1). For example, HLA-E is a low polymorphic, non-classical HLA class I molecule and a major ligand for the inhibitory receptor CD94/NKG2A, which is expressed on a subset of NK cells. Thus, expression of HLA-E on pig endothelial cell at least partially prevents direct human NK cytotoxicity. This finding was confirmed in in vitro studies [79, 80] and in ex vivo perfusion studies of porcine limbs, hearts and lungs with human blood [8, 76, 81]. In addition, decreased proliferation of both human T and NK cells was demonstrated in vitro in response to fibroblasts derived from GTKO pigs expressing HLA-G1+. Furthermore, transplantation of islets from GTKO/HLA-G1+ pigs into diabetic mice resulted in restored normoglycemia [77]. Several other studies have confirmed the partial inhibition of NK cell cytotoxicity, but also of adhesion, by HLA-G1 expressed on pEC in vitro [96–98], including soluble HLA-G1 [99]. Finally, higher levels of protection have been achieved by co-expressing HLA-E and HLA-G1 in vitro assays, primarily by stabilizing HLA-E expression [82, 83]. However, complete protection from xenogeneic NK cytotoxicity has not yet been achieved, probably due to the heterogeneity of the inhibitory NK cell receptor repertoire.
The role of αGal in recognition of porcine target cells by human NK cells was extensively reviewed in 2017 [12]. In short, human NK cytotoxicity against αGalKO pEC in the absence of Abs was equivalent to that in αGal expressing cells. On the other hand, interactions between αGal and the activating NK cell receptor CD161 remains a matter of debate [100–102]. The deletion of αGal might result in the recognition of terminal epitopes and N-acetyllactosamine (NAcLac) [100] by NK cells expressing CD161, however, the significance of such neoantigens needs further investigation. Immortalized pEC with gene KO for the enzymes of the three major xenoAg sugars, GGTA1, CMAH and B4GALNT2, with or without deletion of SLA-I α-chain/β-2, strongly induced degranulation of IL-2-activated human NK cells [103]. The results indicate that other so far unidentified activating ligands of carbohydrate and/or protein nature, are involved in human anti-pig NK cytotoxicity. The question of the exact ligands on porcine cells stays unsolved. However, some of these unidentified ligands can be masked by dextran, an analog of heparan sulfate proteoglycan, which is a key molecule for the anticoagulant and anti-inflammatory properties of the resting (non-activated) endothelium, providing partial protection against human NK cytotoxicity [104].
Human NK cell receptors NKp44 and NKG2D are activated by ligands present in pECs [37] and porcine chondrocytes [105]. Although porcine ULBP1 has been confirmed as a ligand for human NKG2D, its removal did not result in complete inhibition of NK cell cytotoxicity, indicating the potential involvement of additional ligands [106]. Using CRISPR technology, more recent findings demonstrated that pULBP1 gene knockout in porcine endothelial cell lines or transgenic pigs was not sufficient to fully suppress NK cell degranulation and cytotoxicity [84, 85].
Some other alternative and indirect methods of NK cell inhibition were explored in vitro. Controversial data have been reported on the protective effect of FasL expression on porcine endothelial cells [21, 87, 107]. An effective strategy to mitigate macrophage responses is the transgenic expression of CD47 on porcine cells, which acts as a “don’t eat me” signal via the receptor SIRPα and inhibits phagocytosis [108–110]. Of note, CD47 was present in the 10GE pig hearts used in the first pig-to-human xenotransplantations. It appears that it did not entirely prevent macrophage responses, as CD68 staining demonstrated clusters of macrophages on POD34. However, CD47 gene expression in the heart decreased over time, as other transgenes (HO1, CD46, and DAF) also showed lower expression, raising the question of how effective and robust the genetic modifications were [74]. Since NK cells can also express SIRPα they could potentially be inhibited via this pathway. Indeed, expression of CD47 on HLA-deficient target cells inhibited SIRPα-expressing NK cells in vivo in a mouse model and in vitro using a CD47 transduced NK target cell line (K562) [35]. These recent results need to be confirmed in the pig-to-human and pig-to-NHP settings.
Cytotoxic T Lymphocytes
As mentioned above, T cells recognize porcine cells directly restricted by MHC molecules; therefore, elimination of SLA class I expression on pig cells should protect from CD8+ T lymphocyte-mediated lysis. Indeed, cells obtained from engineered pigs knocked down for beta-2 microglobulin (KO B2M) and expressing an SLA-Ilow phenotype were less capable in triggering proliferation of human PBMC in vitro, which was mainly due to the non-responsiveness of CD8+ T cells. However, no difference in cytotoxicity exerted by human PBMC or purified NK cells was observed [86]. Alternatively, as shown in a recent in vitro study, silenced expression of SLA-I and -II on porcine kidney epithelial cells resulted in weak CTL reactivity and cytokine secretion but, surprisingly, did not trigger NK cell degranulation [111]. Therefore, further investigation is required to gain a deeper comprehension of the impact of SLA knockouts or silencing on CTL and NK cell responses. This is crucial to prevent the potential exacerbation of the missing-self problem.
One of the ways to prevent delayed xenograft rejection (DXR) orchestrated by adaptive immunity is the administration of immunosuppressive therapy (see below), including blocking co-stimulatory molecules of T cell – APC interactions (i.e., CD80/CD86-CD28) with CTLA4-Ig (Abatacept) or its affinity-optimized derivative LEA29Y (Belatacept) [112]. To circumvent the drawbacks of systemic immunosuppression, these inhibitory molecules have been expressed transgenically in pig cells with the objective of enabling local protection of the xenograft from CTL [91]. It was shown that serum from LEA29Y [92] and hCTLA4-Ig [90] transgenic pigs reduced the proliferation of T cells in a mixed-lymphocyte reaction compared to wild-type (WT) controls. Another similar approach to protect xenograft is by expression of human programmed death-ligand 1 (hPD-L1) on porcine cells, which diminished human T cell- and NK cell-mediated cytotoxicity and proliferation in vitro [93, 94]. In a recent study, human T cells were perfused through porcine kidneys expressing hPD-L1 ex vivo. T cells adhered similarly to the endothelium and infiltrated the tissue of both WT and transgenic kidneys, but secretion of IFNγ, TNF, granzyme B, and perforin was decreased. The inhibition of T cell activity was confirmed in vitro by co-culturing renal pECs in proliferation and cytotoxicity assays [95]. The expression of inhibitory molecules proved to be quite efficient in both in vitro and ex vivo experiments. However, the confirmation of these findings in vivo remains to be seen.
The Fas/FasL pathway utilized by cytotoxic cells presents another potential target for inhibiting NK and CTL responses. Overexpressing membrane-bound human FasL and a human decoy Fas in pECs led to partial protection from CTL-mediated cytotoxicity [21]. However, protection from NK cell cytotoxicity was not achieved in vitro, in contrast NK cell migration through pECs and chemotaxis of human polymorphonuclear cells were strongly increased by the expression and cleavage of soluble FasL [107]. As an inhibitor of Fas-receptor signaling, overexpressed porcine cellular FLICE inhibitory protein (c-FLIP) in pECs resulted in a significant reduction of CTL-mediated lysis, with up to 75% suppression confirmed in vitro and prolonged graft survival in a rat xenotransplantation model [88].
The question of tolerance induction arises. It would be advantageous to induce tolerance of the innate and adaptive immune systems in order to prevent rejection while maintaining the ability of the immune system to protect the transplant recipient and the graft from infection. One approach to induce recipient tolerance and to avoid heavy immunosuppression could involve porcine thymus transplantation. Studies in a pig-to-humanized mouse model have demonstrated its efficacy in promoting tolerance both in mixed leukocyte reactions [113] and xenograft settings [114]. However, efficient positive selection of human T cells in the porcine thymus remains a challenge [115]. Porcine dendritic cells are likely to be pivotal cells in the initiation of CTL responses [116]. An alternative approach to achieving T-cell tolerance is therefore to use tolerogenic or regulatory dendritic cells (DC), or porcine mesenchymal stromal cells (pMSC) aiming to prevent indirect recognition [54, 117–120].
Co-Stimulation Blockade and Immunosuppressive Regimens
Costimulation blockade to inhibit xenogeneic NK and CTL responses can be achieved by two major approaches: (i) blocking receptor-ligand interactions between human or porcine APC and human T cells, using pharmacological compounds such as Abatacept/Belatacept or antibodies directed against CD40 or CD154, or (ii) by transgenic expression of genes with an inhibitory effect on costimulation in pig endothelial cells (surface expression and secretion) such as hCD152 (CTLA4 or PD-L1). Blocking of pCD86, a ligand of the activating costimulatory receptor CD28, combined with αGal downregulation, resulted in complete protection from human NK cell cytotoxicity [64]. In a previous study, Costa and colleagues demonstrated that the expression of the inhibitory costimulation receptor hCD152 (hCTLA4-Ig) on pECs resulted in the inhibition of T cell and NK cell activation [64]. The same group later developed a pig-to-mouse xenotransplantation model where they implanted pECs transduced with hCD152-hCD59 under the recipients’ kidney capsules. Enhanced survival for up to 5 weeks was observed in modified xenografts compared to the control group [89]. Simultaneous blocking of NK cell activating receptors CD2 and NKG2D resulted in the suppression of NK-mediated killing in vitro [36]. The blockade of the CD40−CD154 co-stimulation pathway using therapeutic monoclonal antibodies was reported to be successful in pig-to-NHP kidney transplantation [121], and in pig-to-NHP corneal transplantation, the xenografts exhibited prolonged survival (more than 933 days) [121]. This outcome was particularly attributable to the reduction in cellular infiltrates, including CTL and macrophages, as well as the diminished levels of cytokines (i.e., IFNγ, TNF, IL-2) [122]. Given that activated NK cells express CD154 (CD40L), and that binding to CD40 provides a positive signal leading to cytotoxicity [65, 123], the blockade of CD154 signaling may also be beneficial in attenuating NK cell responses. However, this hypothesis has yet to be investigated. Concurrently, the simultaneous blockade of CD28 and CD40−CD154 pathways resulted in the complete abrogation of T cell proliferation when co-cultured with porcine APCs in a preliminary report [124]. Conversely, the cross-species interaction of CD154-pCD40 resulted in pEC activation, with the induction of E-selectin (CD62E), VCAM-1 (CD106) and SLA class-II [125].
The use of an immunosuppressive (IS) regimen is essential for prolonging xenograft survival in pig-to-NHP models despite the utilization of genetically modified porcine tissues and organs. For example, IS consisting of cyclosporin A, cyclophosphamide, and methylprednisolone delayed rejection in baboons and rhesus monkeys [68, 126]. Other experiments using hCD46 transgenic pig hearts and liver hDAF (hCD55) xenografts resulted in rejection characterized by infiltrates with CD4+ and CD8+ T cells [69]. Since those experiments were performed without prior T cell depletion, the effects of CTL were detrimental quite early after transplantation. T cell depletion showed benefits for renal graft survival (>400 days) in rhesus macaques [127]. Interestingly, these results were achieved by selected targeting of CD4+ but not CD8+ T cells, which could be explained by the failure of CD8+ T cells to exert their function in the absence of helper T cells. Although this approach initially proves effective, CD8+ T cells proliferate rapidly following T cell depletion therapies, with the majority comprising effector-memory T cells [128]. This was recently confirmed in a pig-to-baboon genetically modified porcine kidney and heart transplantation models [10, 129–131]. In compassionate pig-to-human cardiac xenotransplantation, IS regimen based on pig-to-baboon studies (induction with ATG and anti-CD20, anti-CD40, MMF, tacrolimus) was insufficient to provide long-term survival, presumably due to patients’ comorbidities [74]. Rapamycin, an mTOR-inhibitor exhibits several properties that may confer particular benefit in the context of xenotransplantation, including inhibition of T cell proliferation, promotion of the number of T regulatory cells, prevention of pig graft growth, and anti-inflammatory, anti-viral and anti-cancer effects [132, 133]. Whereas the use of rapamycin, a mTOR pathway blocker used as a maintenance IS treatment, has been observed in vitro to interfere with the xeno-specific T cell proliferative responses to pEC, in addition to modulating the pEC immunogenicity via a PD-1/PD-L1 independent pathway. Under the same conditions, belatacept only partially inhibited xenogeneic T cell proliferation and reduction of CD2 expression [134].
With regard to the impact of IS regimens on NK cells, our findings indicate that a range of NK cell functions can be suppressed by IS drugs (such as methylprednisolone and therapeutic immunoglobulins) in vitro [135]. Recently, clinical trials of a therapeutic CD38 antibody for antibody-mediated rejection in kidney allotransplantation have demonstrated a transient depletion of CD16bright NK cells and improved graft function. These results may potentially be implemented in the context of xenotransplantation [136].
Concluding Remarks and Future Perspectives
In recent years, xenotransplantation has made significant progress, especially with the advent of genetic modifications designed to attenuate hyperacute rejection. Notwithstanding these advances, acute vascular rejection and delayed xenograft rejection continue to present substantial challenges, driven by intricate interactions between the recipient’s innate and adaptive immune systems and the xenograft. Genetic modifications have shown efficiency in mitigating several immune responses, yet they fall short in providing comprehensive protection from NK and CTL-mediated cytotoxicity.
In conclusion, there are multiple pivotal areas within the field of xenotransplantation research that warrant further investigation. First, additional combinations of genetic modifications to porcine tissues should be explored, such as more effective expression of human non-classical MHC class I molecules (HLA-E, HLA-G1) in addition to targeting SLA class I expression, with the aim of better suppressing NK and CTL responses. Of particular interest would also be combinations of other inhibitory pathway transgenes, such as co-expression of HLA-E, CD47, PD-L1, and so forth. Secondly, the utilization of multi-omics analyses will likely offer deeper insights into the immune response at various stages following xenotransplantation, which may ultimately result in the identification of novel therapeutic targets. Thirdly, research into local immunosuppression techniques, such as the expression of human PD-L1 or LEA29Y on xenografts, could help in minimizing systemic immunosuppression and enhancing the long-term viability of xenografts. Future work will likely also explore novel combinations of co-stimulation blockade with organs from transgenic pigs to manage T cell and NK cell activation more effectively. Allotransplantation trials will provide new insights generating strategies for addressing antibody- and cellular-mediated rejection. Another avenue for exploration is the role of glycosylation in cellular responses and the potential of inducing recipient tolerance, for example, through thymus transplantation, thereby reducing the need for lifelong immunosuppression. Ultimately, understanding and addressing the complexities of both innate and adaptive immune responses will be crucial to overcoming the remaining barriers in xenotransplantation and advancing its successful clinical application.
Author Contributions
VG wrote and edited the manuscript GPY conducted several revisions of the manuscript JS conducted several revisions of the manuscript. All authors contributed to the article and approved the submitted version.
Funding
The author(s) declare that financial support was received for the research, authorship, and/or publication of this article. Xenotransplantation research in the laboratory of JS is supported by a Swiss National Science Foundation (SNSF) SINERGIA grant (#CRSII5_198577) and a private foundation.
Conflict of Interest
The salary of GPY was partially funded by an unrestricted grant from CSL Behring.
The remaining authors declare that the research was conducted in the absence of any commercial or financial relationships that could be construed as a potential conflict of interest.
Generative AI Statement
The author(s) declare that no Generative AI was used in the creation of this manuscript.
References
1. Ali, A, Kemter, E, and Wolf, E. Advances in Organ and Tissue Xenotransplantation. Annu Rev Anim Biosci (2024) 12:369–90. doi:10.1146/annurev-animal-021122-102606
2. Sykes, M, and Sachs, DH. Progress in Xenotransplantation: Overcoming Immune Barriers. Nat Rev Nephrol (2022) 18(12):745–61. doi:10.1038/s41581-022-00624-6
3. Cooper, DKC, and Pierson, RN. Milestones on the Path to Clinical Pig Organ Xenotransplantation. Am J Transpl (2023) 23(3):326–35. doi:10.1016/j.ajt.2022.12.023
4. Baumann, BC, Forte, P, Hawley, RJ, Rieben, R, Schneider, MKJ, and Seebach, JD. Lack of Galactose-Alpha-1,3-Galactose Expression on Porcine Endothelial Cells Prevents Complement-Induced Lysis but Not Direct Xenogeneic NK Cytotoxicity. J Immunol (2004) 172(10):6460–7. doi:10.4049/jimmunol.172.10.6460
5. Estrada, JL, Martens, G, Li, P, Adams, A, Newell, KA, Ford, ML, et al. Evaluation of Human and Non-Human Primate Antibody Binding to Pig Cells Lacking GGTA1/CMAH/β4GalNT2 Genes. Xenotransplantation (2015) 22(3):194–202. doi:10.1111/xen.12161
6. Wang, RG, Ruan, M, Zhang, RJ, Chen, L, Li, XX, Fang, B, et al. Antigenicity of Tissues and Organs From GGTA1/CMAH/β4GalNT2 Triple Gene Knockout Pigs. J Biomed Res (2018) 33(4):235–43. doi:10.7555/JBR.32.20180018
7. Bongoni, AK, Kiermeir, D, Jenni, H, Bähr, A, Ayares, D, Klymiuk, N, et al. Complement Dependent Early Immunological Responses During Ex Vivo Xenoperfusion of hCD46/HLA-E Double Transgenic Pig Forelimbs With Human Blood. Xenotransplantation (2014) 21(3):230–43. doi:10.1111/xen.12090
8. Puga Yung, G, Bongoni, AK, Pradier, A, Madelon, N, Papaserafeim, M, Sfriso, R, et al. Release of Pig Leukocytes and Reduced Human NK Cell Recruitment During Ex Vivo Perfusion of HLA-E/Human CD46 Double-Transgenic Pig Limbs With Human Blood. Xenotransplantation (2018) 25(1). doi:10.1111/xen.12357
9. Langin, M, Mayr, T, Reichart, B, Michel, S, Buchholz, S, Guethoff, S, et al. Consistent Success in Life-Supporting Porcine Cardiac Xenotransplantation. Nature (2018) 564(7736):430–3. doi:10.1038/s41586-018-0765-z
10. Eisenson, D, Hisadome, Y, Santillan, M, Iwase, H, Chen, W, Shimizu, A, et al. Consistent Survival in Consecutive Cases of Life-Supporting Porcine Kidney Xenotransplantation Using 10GE Source Pigs. Nat Commun (2024) 15(1):3361. doi:10.1038/s41467-024-47679-6
11. Inverardi, L, Samaja, M, Motterlini, R, Mangili, F, Bender, JR, and Pardi, R. Early Recognition of a Discordant Xenogeneic Organ by Human Circulating Lymphocytes. J Immunol (1992) 149(4):1416–23.
12. Puga Yung, G, Schneider, MKJ, and Seebach, JD. The Role of NK Cells in Pig-To-Human Xenotransplantation. J Immunol Res (2017) 2017:4627384. doi:10.1155/2017/4627384
13. Maeda, A, Kogata, S, Toyama, C, Lo, PC, Okamatsu, C, Yamamoto, R, et al. The Innate Cellular Immune Response in Xenotransplantation. Front Immunol (2022) 13:858604. doi:10.3389/fimmu.2022.858604
14. Lopez, KJ, Cross-Najafi, AA, Farag, K, Obando, B, Thadasina, D, Isidan, A, et al. Strategies to Induce Natural Killer Cell Tolerance in Xenotransplantation. Front Immunol (2022) 13:941880. doi:10.3389/fimmu.2022.941880
15. Gao, H, Zhang, Q, Chen, J, Cooper, DKC, Hara, H, Chen, P, et al. Porcine IL-6, IL-1β, and TNF-α Regulate the Expression of Pro-Inflammatory-Related Genes and Tissue Factor in Human Umbilical Vein Endothelial Cells. Xenotransplantation (2018) 25(5):e12408. doi:10.1111/xen.12408
16. Zhao, Y, Cooper, DKC, Wang, H, Chen, P, He, C, Cai, Z, et al. Potential Pathological Role of Pro-Inflammatory Cytokines (IL-6, TNF-α, and IL-17) in Xenotransplantation. Xenotransplantation (2019) 26(3):e12502. doi:10.1111/xen.12502
17. Birmele, B, Thibault, G, Nivet, H, Gruel, Y, Bardos, P, and Lebranchu, Y. Human Lymphocyte Adhesion to Xenogeneic Porcine Endothelial Cells: Modulation by Human TNF-Alpha and Involvement of VLA-4 and LFA-1. Transpl Immunol (1996) 4(4):265–70. doi:10.1016/s0966-3274(96)80046-3
18. Schneider, MK, Ghielmetti, M, Rhyner, DM, Antsiferova, MA, and Seebach, JD. Human Leukocyte Transmigration Across Galalpha(1,3)Gal-Negative Porcine Endothelium Is Regulated by Human CD18 and CD99. Transplantation (2009) 87(4):491–9. doi:10.1097/TP.0b013e318195fb8d
19. Nasu, K, Whyte, A, Green, SJ, Evans, PC, and Kilshaw, PJ. Alpha-Galactosyl-Mediated Activation of Porcine Endothelial Cells: Studies on CD31 and VE-Cadherin in Adhesion and Signaling. Transplantation (1999) 68(6):861–7. doi:10.1097/00007890-199909270-00020
20. Zheng, L, Ben, LH, Pober, JS, and Bothwell, ALM. Porcine Endothelial Cells, Unlike Human Endothelial Cells, Can Be Killed by Human CTL via Fas Ligand and Cannot Be Protected by Bcl-2. J Immunol (2002) 169(12):6850–5. doi:10.4049/jimmunol.169.12.6850
21. Kawamoto, K, Tanemura, M, Nishida, T, Fukuzawa, M, Ito, T, and Matsuda, H. Significant Inhibition of Human CD8(+) Cytotoxic T Lymphocyte-Mediated Xenocytotoxicity by Overexpression of the Human Decoy Fas Antigen. Transplantation (2006) 81(5):789–96. doi:10.1097/01.tp.0000199266.07237.25
22. Yi, S, Feng, X, Wang, Y, Kay, TW, Wang, Y, and O'Connell, PJ. CD4+ Cells Play a Major Role in Xenogeneic Human Anti-Pig Cytotoxicity Through the Fas/Fas Ligand Lytic Pathway. Transplantation (1999) 67(3):435–43. doi:10.1097/00007890-199902150-00017
23. Berke, G. The Binding and Lysis of Target-Cells by Cytotoxic Lymphocytes - Molecular and Cellular Aspects. Annu Rev Immunol (1994) 12:735–73. doi:10.1146/annurev.iy.12.040194.003511
24. Brincks, EL, Katewa, A, Kucaba, TA, Griffith, TS, and Legge, KL. CD8 T Cells Utilize TRAIL to Control Influenza Virus Infection. J Immunol (2008) 181(7):4918–25. doi:10.4049/jimmunol.181.7.4918
25. Zamai, L, Ahmad, M, Bennett, IM, Azzoni, L, Alnemri, ES, and Perussia, B. Natural Killer (NK) Cell-Mediated Cytotoxicity: Differential Use of TRAIL and Fas Ligand by Immature and Mature Primary Human NK Cells. J Exp Med (1998) 188(12):2375–80. doi:10.1084/jem.188.12.2375
26. Krebs, P, Barnes, MJ, Lampe, K, Whitley, K, Bahjat, KS, Beutler, B, et al. NK-Cell-Mediated Killing of Target Cells Triggers Robust Antigen-Specific T-Cell-Mediated and Humoral Responses. Blood (2009) 113(26):6593–602. doi:10.1182/blood-2009-01-201467
27. Brevig, T, and Kristensen, T. Direct Cytotoxic Response of Human Lymphocytes to Porcine PHA-Lymphoblasts and Lymphocytes. APMIS (1997) 105(4):290–8. doi:10.1111/j.1699-0463.1997.tb00571.x
28. Uzhachenko, RV, and Shanker, A. CD8(+) T Lymphocyte and NK Cell Network: Circuitry in the Cytotoxic Domain of Immunity. Front Immunol (2019) 10:1906. doi:10.3389/fimmu.2019.01906
29. Park, EM, Lee, H, Kang, HJ, Oh, KB, Kim, JS, Chee, HK, et al. Early Interferon-Gamma Response in Nonhuman Primate Recipients of Solid-Organ Xenotransplantation. Transpl Proc (2021) 53(10):3093–100. doi:10.1016/j.transproceed.2021.09.028
30. Pan, W, Zhang, W, Zheng, B, Camellato, BR, Stern, J, Lin, Z, et al. Cellular Dynamics in Pig-To-Human Kidney Xenotransplantation. Med (2024) 5(8):1016–29 e4. doi:10.1016/j.medj.2024.05.003
31. Sfriso, R, Zhang, S, Bichsel, CA, Steck, O, Despont, A, Guenat, OT, et al. 3D Artificial Round Section Micro-Vessels to Investigate Endothelial Cells Under Physiological Flow Conditions. Sci Rep (2018) 8(1):5898. doi:10.1038/s41598-018-24273-7
32. Seebach, JD, Yamada, K, McMorrow, IM, Sachs, DH, and DerSimonian, H. Xenogeneic Human Anti-Pig Cytotoxicity Mediated by Activated Natural Killer Cells. Xenotransplantation (1996) 3(2):188–97. doi:10.1111/j.1399-3089.1996.tb00137.x
33. Watier, H, Guillaumin, JM, Vallée, I, Thibault, G, Gruel, Y, Lebranchu, Y, et al. Human NK Cell-Mediated Direct and IgG-Dependent Cytotoxicity against Xenogeneic Porcine Endothelial Cells. Transpl Immunol (1996) 4(4):293–9. doi:10.1016/s0966-3274(96)80050-5
34. Sullivan, JA, Oettinger, HF, Sachs, DH, and Edge, AS. Analysis of Polymorphism in Porcine MHC Class I Genes: Alterations in Signals Recognized by Human Cytotoxic Lymphocytes. J Immunol (1997) 159(5):2318–26.
35. Deuse, T, Hu, X, Agbor-Enoh, S, Jang, MK, Alawi, M, Saygi, C, et al. The SIRPα-CD47 Immune Checkpoint in NK Cells. J Exp Med (2021) 218(3):e20200839. doi:10.1084/jem.20200839
36. Kim, TJ, Kim, N, Kim, EO, Choi, JR, Bluestone, JA, and Lee, KM. Suppression of Human Anti-Porcine Natural Killer Cell Xenogeneic Responses by Combinations of Monoclonal Antibodies Specific to CD2 and NKG2D and Extracellular Signal-Regulated Kinase Kinase Inhibitor. Immunology (2010) 130(4):545–55. doi:10.1111/j.1365-2567.2010.03253.x
37. Forte, P, Lilienfeld, BG, Baumann, BC, and Seebach, JD. Human NK Cytotoxicity Against Porcine Cells Is Triggered by NKp44 and NKG2D. J Immunol (2005) 175(8):5463–70. doi:10.4049/jimmunol.175.8.5463
38. Brossay, A, Hubé, F, Moreau, T, Bardos, P, and Watier, H. Porcine CD58: CDNA Cloning and Molecular Dissection of the Porcine CD58-Human CD2 Interface. Biochem Biophys Res Commun (2003) 309(4):992–8. doi:10.1016/j.bbrc.2003.08.099
39. Binder, C, Cvetkovski, F, Sellberg, F, Berg, S, Paternina Visbal, H, Sachs, DH, et al. CD2 Immunobiology. Front Immunol (2020) 11:1090. doi:10.3389/fimmu.2020.01090
40. Crosby, K, Yatko, C, Dersimonian, H, Pan, L, and Edge, ASB. A Novel Monoclonal Antibody Inhibits the Immune Response of Human Cells against Porcine Cells: Identification of a Porcine Antigen Homologous to CD58. Transplantation (2004) 77(8):1288–94. doi:10.1097/01.tp.0000120377.57543.d8
41. Parodi, M, Favoreel, H, Candiano, G, Gaggero, S, Sivori, S, Mingari, MC, et al. NKp44-NKp44 Ligand Interactions in the Regulation of Natural Killer Cells and Other Innate Lymphoid Cells in Humans. Front Immunol (2019) 10:719. doi:10.3389/fimmu.2019.00719
42. Padoan, B, Casar, C, Krause, J, Schultheiss, C, Baumdick, ME, Niehrs, A, et al. NKp44/HLA-DP-Dependent Regulation of CD8 Effector T Cells by NK Cells. Cell Rep (2024) 43(4):114089. doi:10.1016/j.celrep.2024.114089
43. Rosenstock, P, and Kaufmann, T. Sialic Acids and Their Influence on Human NK Cell Function. Cells (2021) 10(2):263. doi:10.3390/cells10020263
44. Horvath-Arcidiacono, JA, and Bloom, ET. Characterization of Human Killer Cell Reactivity against Porcine Target Cells: Differential Modulation by Cytokines. Xenotransplantation (2001) 8(1):62–74. doi:10.1034/j.1399-3089.2001.00078.x
45. Yamada, K, Sachs, DH, and DerSimonian, H. Human Anti-Porcine Xenogeneic T Cell Response. Evidence for Allelic Specificity of Mixed Leukocyte Reaction and for Both Direct and Indirect Pathways of Recognition. J Immunol (1995) 155(11):5249–56. doi:10.4049/jimmunol.155.11.5249
46. Scalea, J, Hanecamp, I, Robson, SC, and Yamada, K. T-Cell-Mediated Immunological Barriers to Xenotransplantation. Xenotransplantation (2012) 19(1):23–30. doi:10.1111/j.1399-3089.2011.00687.x
47. Zhou, Q, Li, T, Wang, K, Zhang, Q, Geng, Z, Deng, S, et al. Current Status of Xenotransplantation Research and the Strategies for Preventing Xenograft Rejection. Front Immunol (2022) 13:928173. doi:10.3389/fimmu.2022.928173
48. Vadori, M, and Cozzi, E. The Immunological Barriers to Xenotransplantation. Tissue Antigens (2015) 86(4):239–53. doi:10.1111/tan.12669
49. Dorling, A, Lombardi, G, Binns, R, and Lechler, RI. Detection of Primary Direct and Indirect Human Anti-Porcine T Cell Responses Using a Porcine Dendritic Cell Population. Eur J Immunol (1996) 26(6):1378–87. doi:10.1002/eji.1830260630
50. Rogers, NJ, Jackson, IM, Jordan, WJ, Hawadle, MA, Dorling, A, and Lechler, RI. Cross-Species Costimulation: Relative Contributions of CD80, CD86, and CD40. Transplantation (2003) 75(12):2068–76. doi:10.1097/01.TP.0000069100.67646.08
51. Diaz, LA, Pai, R, Endres, J, Anthony, P, Duzyj, C, Bishu, S, et al. Xenogeneic Cells and Superantigen Induce Human T-Cell Activation in the Absence of T-Cell Recognition of Xenoantigen. J Lab Clin Med (2003) 142(3):149–57. doi:10.1016/S0022-2143(03)00101-X
52. Li, S, Anwar, IJ, Canning, AJ, Vo-Dinh, T, Kirk, AD, and Xu, H. Xenorecognition and Costimulation of Porcine Endothelium-Derived Extracellular Vesicles in Initiating Human Porcine-Specific T Cell Immune Responses. Am J Transpl (2023) 23(7):904–19. doi:10.1016/j.ajt.2023.04.006
53. Murray, AG, Khodadoust, MM, Pober, JS, and Bothwell, AL. Porcine Aortic Endothelial Cells Activate Human T Cells: Direct Presentation of MHC Antigens and Costimulation by Ligands for Human CD2 and CD28. Immunity (1994) 1(1):57–63. doi:10.1016/1074-7613(94)90009-4
54. Park, CS, Im, SA, Song, S, Kim, K, and Lee, CK. Identification of HLA-A2-Restricted Immunogenic Peptides Derived From a Xenogenic Porcine Major Histocompatibility Complex. Xenotransplantation (2014) 21(5):465–72. doi:10.1111/xen.12119
55. Vallee, I, Guillaumin, JM, Thibault, G, Gruel, Y, Lebranchu, Y, Bardos, P, et al. Human T Lymphocyte Proliferative Response to Resting Porcine Endothelial Cells Results From an HLA-Restricted, IL-10-Sensitive, Indirect Presentation Pathway But Also Depends on Endothelial-Specific Costimulatory Factors. J Immunol (1998) 161(4):1652–8.
56. Lalain, S, Chaillous, L, Gouin, E, and Saï, P. Intensity and Mechanisms of In Vitro Xenorecognition of Adult Pig Pancreatic Islet Cells by CD4+ and CD8+ Lymphocytes From Type I Diabetic or Healthy Subjects. Diabetologia (1999) 42(3):330–5. doi:10.1007/s001250051159
57. Xu, XC, Naziruddin, B, Sasaki, H, Smith, DM, and Mohanakumar, T. Allele-Specific and Peptide-Dependent Recognition of Swine Leukocyte Antigen Class I by Human Cytotoxic T-Cell Clones. Transplantation (1999) 68(4):473–9. doi:10.1097/00007890-199908270-00005
58. Ramachandran, S, Jaramillo, A, Xu, XC, McKane, BW, Chapman, WC, and Mohanakumar, T. Human Immune Responses to Porcine Endogenous Retrovirus-Derived Peptides Presented Naturally in the Context of Porcine and Human Major Histocompatibility Complex Class I Molecules: Implications in Xenotransplantation of Porcine Organs. Transplantation (2004) 77(10):1580–8. doi:10.1097/01.tp.0000122220.61309.1d
59. Yamada, K, Seebach, JD, DerSimonian, H, and Sachs, DH. Human Anti-Pig T-Cell Mediated Cytotoxicity. Xenotransplantation (1996) 3(2):179–87. doi:10.1111/j.1399-3089.1996.tb00136.x
60. Omilusik, KD, and Goldrath, AW. The Origins of Memory T Cells. Nature (2017) 552(7685):337–9. doi:10.1038/d41586-017-08280-8
61. Maher, SE, Karmann, K, Min, W, Hughes, CC, Pober, JS, and Bothwell, AL. Porcine Endothelial CD86 Is a Major Costimulator of Xenogeneic Human T Cells: Cloning, Sequencing, and Functional Expression in Human Endothelial Cells. J Immunol (1996) 157(9):3838–44.
62. Davis, TA, Craighead, N, Williams, AJ, Scadron, A, June, CH, and Lee, KP. Primary Porcine Endothelial Cells Express Membrane-Bound B7-2 (CD86) and a Soluble Factor that Co-Stimulate Cyclosporin A-Resistant and CD28-Dependent Human T Cell Proliferation. Int Immunol (1996) 8(7):1099–111. doi:10.1093/intimm/8.7.1099
63. Galea-Lauri, J, Darling, D, Gan, SU, Krivochtchapov, L, Kuiper, M, Gäken, J, et al. Expression of a Variant of CD28 on a Subpopulation of Human NK Cells: Implications for B7-Mediated Stimulation of NK Cells. J Immunol (1999) 163(1):62–70.
64. Costa, C, Barber, DF, and Fodor, WL. Human NK Cell-Mediated Cytotoxicity Triggered by CD86 and Gal Alpha 1,3-Gal Is Inhibited in Genetically Modified Porcine Cells. J Immunol (2002) 168(8):3808–16. doi:10.4049/jimmunol.168.8.3808
65. Carbone, E, Ruggiero, G, Terrazzano, G, Palomba, C, Manzo, C, Fontana, S, et al. A New Mechanism of NK Cell Cytotoxicity Activation: The CD40-CD40 Ligand Interaction. J Exp Med (1997) 185(12):2053–60. doi:10.1084/jem.185.12.2053
66. Tay, NQ, Lee, DCP, Chua, YL, Prabhu, N, Gascoigne, NRJ, and Kemeny, DM. CD40L Expression Allows CD8(+) T Cells to Promote Their Own Expansion and Differentiation through Dendritic Cells. Front Immunol (2017) 8:1484. doi:10.3389/fimmu.2017.01484
67. Wilhite, T, Ezzelarab, C, Hara, H, Long, C, Ayares, D, Cooper, DKC, et al. The Effect of Gal Expression on Pig Cells on the Human T-Cell Xenoresponse. Xenotransplantation (2012) 19(1):56–63. doi:10.1111/j.1399-3089.2011.00691.x
68. Ashton-Chess, J, Roussel, JC, Manez, R, Ruiz, C, Moreau, A, Cozzi, E, et al. Cellular Participation in Delayed Xenograft Rejection of hCD55 Transgenic Pig Hearts by Baboons. Xenotransplantation (2003) 10(5):446–53. doi:10.1034/j.1399-3089.2003.00018.x
69. Davila, E, Byrne, GW, LaBreche, PT, McGregor, HCJ, Schwab, AK, Davies, WR, et al. T-Cell Responses during Pig-To-Primate Xenotransplantation. Xenotransplantation (2006) 13(1):31–40. doi:10.1111/j.1399-3089.2005.00258.x
70. Soderlund, J, Wennberg, L, Castaños-Velez, E, Biberfeld, P, Zhu, S, Tibell, A, et al. Fetal Porcine Islet-Like Cell Clusters Transplanted to Cynomolgus Monkeys: An Immunohistochemical Study. Transplantation (1999) 67(6):784–91. doi:10.1097/00007890-199903270-00002
71. Kirchhof, N, Shibata, S, Wijkstrom, M, Kulick, DM, Salerno, CT, Clemmings, SM, et al. Reversal of Diabetes in Non-Immunosuppressed Rhesus Macaques by Intraportal Porcine Islet Xenografts Precedes Acute Cellular Rejection. Xenotransplantation (2004) 11(5):396–407. doi:10.1111/j.1399-3089.2004.00157.x
72. Stone, KR, Walgenbach, AW, Abrams, JT, Nelson, J, Gillett, N, and Galili, U. Porcine and Bovine Cartilage Transplants in Cynomolgus Monkey: I. A Model for Chronic Xenograft Rejection. Transplantation (1997) 63(5):640–5. doi:10.1097/00007890-199703150-00005
73. Loupy, A, Goutaudier, V, Giarraputo, A, Mezine, F, Morgand, E, Robin, B, et al. Immune Response after Pig-To-Human Kidney Xenotransplantation: A Multimodal Phenotyping Study. Lancet (2023) 402(10408):1158–69. doi:10.1016/S0140-6736(23)01349-1
74. Mohiuddin, MM, Singh, AK, Scobie, L, Goerlich, CE, Grazioli, A, Saharia, K, et al. Graft Dysfunction in Compassionate Use of Genetically Engineered Pig-To-Human Cardiac Xenotransplantation: A Case Report. Lancet (2023) 402(10399):397–410. doi:10.1016/S0140-6736(23)00775-4
75. Schmauch, E, Piening, B, Mohebnasab, M, Xia, B, Zhu, C, Stern, J, et al. Integrative Multi-Omics Profiling in Human Decedents Receiving Pig Heart Xenografts. Nat Med (2024) 30(5):1448–60. doi:10.1038/s41591-024-02972-1
76. Abicht, JM, Sfriso, R, Reichart, B, Längin, M, Gahle, K, Puga Yung, GL, et al. Multiple Genetically Modified GTKO/hCD46/HLA-E/hβ2-mg Porcine Hearts Are Protected From Complement Activation and Natural Killer Cell Infiltration during Ex Vivo Perfusion With Human Blood. Xenotransplantation (2018) 25(5):e12390. doi:10.1111/xen.12390
77. Rao, JS, Hosny, N, Kumbha, R, Naqvi, RA, Singh, A, Swanson, Z, et al. HLA-G1(+) Expression in GGTA1KO Pigs Suppresses Human and Monkey Anti-Pig T, B and NK Cell Responses. Front Immunol (2021) 12:730545. doi:10.3389/fimmu.2021.730545
78. Seebach, JD, Comrack, C, Germana, S, LeGuern, C, Sachs, DH, and DerSimonian, H. HLA-Cw3 Expression on Porcine Endothelial Cells Protects against Xenogeneic Cytotoxicity Mediated by a Subset of Human NK Cells. J Immunol (1997) 159(7):3655–61.
79. Forte, P, Baumann, BC, Weiss, EH, and Seebach, JD. HLA-E Expression on Porcine Cells: Protection from Human NK Cytotoxicity Depends on Peptide Loading. Am J Transpl (2005) 5(9):2085–93. doi:10.1111/j.1600-6143.2005.00987.x
80. Weiss, EH, Lilienfeld, BG, Müller, S, Müller, E, Herbach, N, Kessler, B, et al. HLA-E/Human Beta2-Microglobulin Transgenic Pigs: Protection Against Xenogeneic Human Anti-Pig Natural Killer Cell Cytotoxicity. Transplantation (2009) 87(1):35–43. doi:10.1097/TP.0b013e318191c784
81. Laird, CT, Burdorf, L, French, BM, Kubicki, N, Cheng, X, Braileanu, G, et al. Transgenic Expression of Human Leukocyte Antigen-E Attenuates GalKO.hCD46 Porcine Lung Xenograft Injury. Xenotransplantation (2017) 24(2). doi:10.1111/xen.12294
82. Cross-Najafi, AA, Farag, K, Isidan, A, Li, W, Zhang, W, Lin, Z, et al. Co-Expression of HLA-E and HLA-G on Genetically Modified Porcine Endothelial Cells Attenuates Human NK Cell-Mediated Degranulation. Front Immunol (2023) 14:1217809. doi:10.3389/fimmu.2023.1217809
83. Matsunami, K, Miyagawa, S, Nakai, R, Yamada, M, and Shirakura, R. Modulation of the Leader Peptide Sequence of the HLA-E Gene Up-Regulates its Expression and Down-Regulates Natural Killer Cell-Mediated Swine Endothelial Cell Lysis. Transplantation (2002) 73(10):1582–9. doi:10.1097/00007890-200205270-00010
84. Joanna, Z, Magdalena, H, Agnieszka, NT, Jacek, J, Ryszard, S, Zdzisław, S, et al. The Production of UL16-Binding Protein 1 Targeted Pigs Using CRISPR Technology. 3 Biotech (2018) 8(1):70. doi:10.1007/s13205-018-1107-4
85. Lopez, KJ, Spence, JP, Li, W, Zhang, W, Wei, B, Cross-Najafi, AA, et al. Porcine UL-16 Binding Protein 1 Is Not a Functional Ligand for the Human Natural Killer Cell Activating Receptor NKG2D. Cells (2023) 12(22):2587. doi:10.3390/cells12222587
86. Hein, R, Sake, HJ, Pokoyski, C, Hundrieser, J, Brinkmann, A, Baars, W, et al. Triple (GGTA1, CMAH, B2M) Modified Pigs Expressing an SLA Class I(low) Phenotype-Effects on Immune Status and Susceptibility to Human Immune Responses. Am J Transpl (2020) 20(4):988–98. doi:10.1111/ajt.15710
87. Kawamoto, K, Tanemura, M, Ito, T, Deguchi, T, Machida, T, Nishida, T, et al. Prolonged Survival of Pig Islets Xenograft by Adenovirus-Mediated Expression of Either the Membrane-Bound Human FasL or the Human Decoy Fas Antigen Gene. Xenotransplantation (2008) 15(5):333–43. doi:10.1111/j.1399-3089.2008.00490.x
88. Tanemura, M, Saga, A, Kawamoto, K, Deguchi, T, Machida, T, Nishida, T, et al. In vitro And In Vivo Prevention of Human CD8+ CTL-Mediated Xenocytotoxicity by Pig C-FLIP Expression in Porcine Endothelial Cells. Am J Transpl (2008) 8(2):288–97. doi:10.1111/j.1600-6143.2007.02077.x
89. Costa, C, Pizzolato, MC, Shen, Y, Wang, Y, and Fodor, WL. CD86 Blockade in Genetically Modified Porcine Cells Delays Xenograft Rejection by Inhibiting T-Cell and NK-Cell Activation. Cel Transpl (2004) 13(1):75–87. doi:10.3727/000000004772664923
90. Wang, Y, Yang, HQ, Jiang, W, Fan, NN, Zhao, BT, Ou-Yang, Z, et al. Transgenic Expression of Human Cytoxic T-Lymphocyte Associated Antigen4-Immunoglobulin (hCTLA4Ig) by Porcine Skin for Xenogeneic Skin Grafting. Transgenic Res (2015) 24(2):199–211. doi:10.1007/s11248-014-9833-9
91. Klymiuk, N, van Buerck, L, Bähr, A, Offers, M, Kessler, B, Wuensch, A, et al. Xenografted Islet Cell Clusters From INSLEA29Y Transgenic Pigs Rescue Diabetes and Prevent Immune Rejection in Humanized Mice. Diabetes (2012) 61(6):1527–32. doi:10.2337/db11-1325
92. Bahr, A, Käser, T, Kemter, E, Gerner, W, Kurome, M, Baars, W, et al. Ubiquitous LEA29Y Expression Blocks T Cell Co-Stimulation But Permits Sexual Reproduction in Genetically Modified Pigs. PLoS One (2016) 11(5):e0155676. doi:10.1371/journal.pone.0155676
93. Plege, A, Borns, K, Beer, L, Baars, W, Klempnauer, J, and Schwinzer, R. Downregulation of Cytolytic Activity of Human Effector Cells by Transgenic Expression of Human PD-Ligand-1 on Porcine Target Cells. Transpl Int (2010) 23(12):1293–300. doi:10.1111/j.1432-2277.2010.01130.x
94. Buermann, A, Petkov, S, Petersen, B, Hein, R, Lucas-Hahn, A, Baars, W, et al. Pigs Expressing the Human Inhibitory Ligand PD-L1 (CD 274) Provide a New Source of Xenogeneic Cells and Tissues With Low Immunogenic Properties. Xenotransplantation (2018) 25(5):e12387. doi:10.1111/xen.12387
95. Schmalkuche, K, Rother, T, Besli, S, Schwinzer, R, Blasczyk, R, Petersen, B, et al. Human PD-L1 Overexpression Decreases Xenogeneic Human T-Cell Immune Responses Towards Porcine Kidneys. Front Immunol (2024) 15:1279050. doi:10.3389/fimmu.2024.1279050
96. Forte, P, Matter-Reissmann, UB, Strasser, M, Schneider, MK, and Seebach, JD. Porcine Aortic Endothelial Cells Transfected with HLA-G Are Partially Protected from Xenogeneic Human NK Cytotoxicity. Hum Immunol (2000) 61(11):1066–73. doi:10.1016/s0198-8859(00)00202-0
97. Wang, SS, Han, JY, Wu, XW, Cao, RH, Qi, HG, Xia, ZX, et al. A Study of HLA-G1 Protection of Porcine Endothelial Cells Against Human NK Cell Cytotoxicity. Transpl Proc (2004) 36(8):2473–4. doi:10.1016/j.transproceed.2004.08.048
98. Matsunami, K, Miyagawa, S, Nakai, R, Murase, A, and Shirakura, R. The Possible Use of HLA-G1 and G3 in the Inhibition of NK Cell-Mediated Swine Endothelial Cell Lysis. Clin Exp Immunol (2001) 126(1):165–72. doi:10.1046/j.1365-2249.2001.01622.x
99. Zeng, MH, Fang, CY, Wang, SS, Zhu, M, Xie, L, Li, R, et al. A Study of Soluble HLA-G1 Protecting Porcine Endothelial Cells against Human Natural Killer Cell-Mediated Cytotoxicity. Transpl Proc (2006) 38(10):3312–4. doi:10.1016/j.transproceed.2006.10.179
100. Christiansen, D, Mouhtouris, E, Milland, J, Zingoni, A, Santoni, A, and Sandrin, MS. Recognition of a Carbohydrate Xenoepitope by Human NKRP1A (CD161). Xenotransplantation (2006) 13(5):440–6. doi:10.1111/j.1399-3089.2006.00332.x
101. Miyagawa, S, Takeishi, S, Yamamoto, A, Ikeda, K, Matsunari, H, Yamada, M, et al. Survey of Glycoantigens in Cells From Alpha1-3galactosyltransferase Knockout Pig Using a Lectin Microarray. Xenotransplantation (2010) 17(1):61–70. doi:10.1111/j.1399-3089.2009.00565.x
102. Miyagawa, S, Ueno, T, Nagashima, H, Takama, Y, and Fukuzawa, M. Carbohydrate Antigens. Curr Opin Organ Transpl (2012) 17(2):174–9. doi:10.1097/MOT.0b013e3283508189
103. Li, P, Walsh, JR, Lopez, K, Isidan, A, Zhang, W, Chen, AM, et al. Genetic Engineering of Porcine Endothelial Cell Lines for Evaluation of Human-To-Pig Xenoreactive Immune Responses. Sci Rep (2021) 11(1):13131. doi:10.1038/s41598-021-92543-y
104. Laumonier, T, Walpen, AJ, Maurus, CF, Mohacsi, PJ, Matozan, KM, Korchagina, EY, et al. Dextran Sulfate Acts as an Endothelial Cell Protectant and Inhibits Human Complement and Natural Killer Cell-Mediated Cytotoxicity Against Porcine Cells. Transplantation (2003) 76(5):838–43. doi:10.1097/01.TP.0000078898.28399.0A
105. Sommaggio, R, Cohnen, A, Watzl, C, and Costa, C. Multiple Receptors Trigger Human NK Cell-Mediated Cytotoxicity Against Porcine Chondrocytes. J Immunol (2012) 188(5):2075–83. doi:10.4049/jimmunol.1100433
106. Lilienfeld, BG, Garcia-Borges, C, Crew, MD, and Seebach, JD. Porcine UL16-Binding Protein 1 Expressed on the Surface of Endothelial Cells Triggers Human NK Cytotoxicity Through NKG2D. J Immunol (2006) 177(4):2146–52. doi:10.4049/jimmunol.177.4.2146
107. Matter-Reissmann, UB, Sonntag, KC, Gilli, UO, Leguern, C, Schneider, MKJ, and Seebach, JD. Human Fas-Ligand Expression on Porcine Endothelial Cells Does Not Protect against Xenogeneic Natural Killer Cytotoxicity. Xenotransplantation (2004) 11(1):43–52. doi:10.1111/j.1399-3089.2004.00081.x
108. Ide, K, Wang, H, Tahara, H, Liu, J, Wang, X, Asahara, T, et al. Role for CD47-SIRPalpha Signaling in Xenograft Rejection by Macrophages. Proc Natl Acad Sci U S A (2007) 104(12):5062–6. doi:10.1073/pnas.0609661104
109. Wang, H, VerHalen, J, Madariaga, ML, Xiang, S, Wang, S, Lan, P, et al. Attenuation of Phagocytosis of Xenogeneic Cells by Manipulating CD47. Blood (2007) 109(2):836–42. doi:10.1182/blood-2006-04-019794
110. Hosny, N, Matson, AW, Kumbha, R, Steinhoff, M, Sushil Rao, J, El-Abaseri, TB, et al. 3'UTR Enhances hCD47 Cell Surface Expression, Self-Signal Function, and Reduces ER Stress in Porcine Fibroblasts. Xenotransplantation (2021) 28(1):e12641. doi:10.1111/xen.12641
111. Schmalkuche, K, Schwinzer, R, Wenzel, N, Valdivia, E, Petersen, B, Blasczyk, R, et al. Downregulation of Swine Leukocyte Antigen Expression Decreases the Strength of Xenogeneic Immune Responses towards Renal Proximal Tubular Epithelial Cells. Int J Mol Sci (2023) 24(16):12711. doi:10.3390/ijms241612711
112. Wolf, E, Kemter, E, Klymiuk, N, and Reichart, B. Genetically Modified Pigs as Donors of Cells, Tissues, and Organs for Xenotransplantation. Anim Front (2019) 9(3):13–20. doi:10.1093/af/vfz014
113. Shimizu, I, Fudaba, Y, Shimizu, A, Yang, YG, and Sykes, M. Comparison of Human T Cell Repertoire Generated in Xenogeneic Porcine and Human Thymus Grafts. Transplantation (2008) 86(4):601–10. doi:10.1097/TP.0b013e318182d47a
114. Kalscheuer, H, Onoe, T, Dahmani, A, Li, HW, Hölzl, M, Yamada, K, et al. Xenograft Tolerance and Immune Function of Human T Cells Developing in Pig Thymus Xenografts. J Immunol (2014) 192(7):3442–50. doi:10.4049/jimmunol.1302886
115. Nauman, G, Borsotti, C, Danzl, N, Khosravi-Maharlooei, M, Li, HW, Chavez, E, et al. Reduced Positive Selection of a Human TCR in a Swine Thymus Using a Humanized Mouse Model for Xenotolerance Induction. Xenotransplantation (2020) 27(1):e12558. doi:10.1111/xen.12558
116. Layton, DS, Bean, AG, Dodge, NM, Strom, ADG, Sandrin, MS, and Ierino, FL. Differential Cytokine Expression and Regulation of Human Anti-Pig Xenogeneic Responses by Modified Porcine Dendritic Cells. Xenotransplantation (2008) 15(4):257–67. doi:10.1111/j.1399-3089.2008.00477.x
117. Dhodapkar, MV, Steinman, RM, Krasovsky, J, Munz, C, and Bhardwaj, N. Antigen-Specific Inhibition of Effector T Cell Function in Humans after Injection of Immature Dendritic Cells. J Exp Med (2001) 193(2):233–8. doi:10.1084/jem.193.2.233
118. Taner, T, Hackstein, H, Wang, Z, Morelli, AE, and Thomson, AW. Rapamycin-Treated, Alloantigen-Pulsed Host Dendritic Cells Induce Ag-Specific T Cell Regulation and Prolong Graft Survival. Am J Transpl (2005) 5(2):228–36. doi:10.1046/j.1600-6143.2004.00673.x
119. Madelon, N, Puga Yung, GL, and Seebach, JD. Human Anti-Pig NK Cell and CD8(+) T-Cell Responses in the Presence of Regulatory Dendritic Cells. Xenotransplantation (2016) 23(6):479–89. doi:10.1111/xen.12279
120. Li, J, Andreyev, O, Chen, M, Marco, M, Iwase, H, Long, C, et al. Human T Cells Upregulate CD69 After Coculture With Xenogeneic Genetically-Modified Pig Mesenchymal Stromal Cells. Cell Immunol (2013) 285(1-2):23–30. doi:10.1016/j.cellimm.2013.08.004
121. Cooper, DKC, Foote, JB, Javed, M, Nguyen, HQ, Bikhet, MH, Hansen-Estruch, C, et al. Initial Evidence that Blockade of the CD40/CD154 Costimulation Pathway Alone Is Sufficient as Maintenance Therapy in Xenotransplantation. Xenotransplantation (2021) 28(6):e12721. doi:10.1111/xen.12721
122. Choi, HJ, Lee, JJ, Kim, DH, Kim, MK, Lee, HJ, Ko, AY, et al. Blockade of CD40-CD154 Costimulatory Pathway Promotes Long-Term Survival of Full-Thickness Porcine Corneal Grafts in Nonhuman Primates: Clinically Applicable Xenocorneal Transplantation. Am J Transpl (2015) 15(3):628–41. doi:10.1111/ajt.13057
123. Terrazzano, G, Zanzi, D, Palomba, C, Carbone, E, Grimaldi, S, Pisanti, S, et al. Differential Involvement of CD40, CD80, and Major Histocompatibility Complex Class I Molecules in Cytotoxicity Induction and Interferon-Gamma Production by Human Natural Killer Effectors. J Leukoc Biol (2002) 72(2):305–11.
124. Popma, SH, Krasinskas, AM, Kreisel, D, Szeto, W, McLean, AD, Moore, JS, et al. Simultaneous Blockade of B7-CD28 and CD40-CD40L Costimulation Eliminates the Direct Xenorestricted Human Anti-Porcine T-Cell Response. Transpl Proc (2001) 33(1-2):767–9. doi:10.1016/s0041-1345(00)02245-4
125. Rushworth, SA, Bravery, CA, and Thompson, S. Human CD154 Induces Activation of Porcine Endothelial Cells and Up-Regulation of MHC Class II Expression. Transplantation (2001) 72(1):127–32. doi:10.1097/00007890-200107150-00025
126. Chen, D, Cao, R, Guo, H, Chen, G, Wang, X, Shen, S, et al. Pathogenesis and Pathology of Delayed Xenograft Rejection in Pig-To-Rhesus Monkey Cardiac Transplantation. Transpl Proc (2004) 36(8):2480–2. doi:10.1016/j.transproceed.2004.09.003
127. Kim, SC, Mathews, DV, Breeden, CP, Higginbotham, LB, Ladowski, J, Martens, G, et al. Long-Term Survival of Pig-To-Rhesus Macaque Renal Xenografts Is Dependent on CD4 T Cell Depletion. Am J Transpl (2019) 19(8):2174–85. doi:10.1111/ajt.15329
128. Zwang, NA, and Turka, LA. Homeostatic Expansion as a Barrier to Lymphocyte Depletion Strategies. Curr Opin Organ Transpl (2014) 19(4):357–62. doi:10.1097/MOT.0000000000000096
129. Jagdale, A, Nguyen, H, Iwase, H, Foote, JB, Yamamoto, T, Javed, M, et al. T and B Lymphocyte Dynamics After Genetically-Modified Pig-To-Baboon Kidney Xenotransplantation With an Anti-CD40mAb-Based Immunosuppressive Regimen. Transpl Immunol (2022) 71:101545. doi:10.1016/j.trim.2022.101545
130. Bender, M, Abicht, JM, Reichart, B, Neumann, E, Radan, J, Mokelke, M, et al. Combination of Anti-CD40 and Anti-CD40L Antibodies as Co-Stimulation Blockade in Preclinical Cardiac Xenotransplantation. Biomedicines (2024) 12(8):1927. doi:10.3390/biomedicines12081927
131. Mohiuddin, MM, Goerlich, CE, Singh, AK, Zhang, T, Tatarov, I, Lewis, B, et al. Progressive Genetic Modifications of Porcine Cardiac Xenografts Extend Survival to 9 Months. Xenotransplantation (2022) 29(3):e12744. doi:10.1111/xen.12744
132. Maenaka, A, Kinoshita, K, Hara, H, and Cooper, DKC. The Case for the Therapeutic Use of Mechanistic/Mammalian Target of Rapamycin (mTOR) Inhibitors in Xenotransplantation. Xenotransplantation (2023) 30(3):e12802. doi:10.1111/xen.12802
133. Zhang, M, Feng, H, Du, J, Chen, S, Zhu, L, Wang, Y, et al. Comparative Inhibitory Effects of Tacrolimus, Cyclosporine, and Rapamycin on Human Anti-Pig Xenogeneic Mixed Lymphocyte Reactions. Xenotransplantation (2024) 31(4):e12876. doi:10.1111/xen.12876
134. Li, S, Xu, H, and Kirk, AD. Modulation of Xenogeneic T-Cell Proliferation by B7 and mTOR Blockade of T Cells and Porcine Endothelial Cells. Transplantation (2022) 106(5):950–62. doi:10.1097/TP.0000000000003920
135. Pradier, A, Papaserafeim, M, Li, N, Rietveld, A, Kaestel, C, Gruaz, L, et al. Small-Molecule Immunosuppressive Drugs and Therapeutic Immunoglobulins Differentially Inhibit NK Cell Effector Functions In Vitro. Front Immunol (2019) 10:556. doi:10.3389/fimmu.2019.00556
Keywords: porcine endothelium, NK cells, CD8+ T cells, xenograft rejection, cytotoxicity
Citation: Galdina V, Puga Yung GL and Seebach JD (2025) Cytotoxic Responses Mediated by NK Cells and Cytotoxic T Lymphocytes in Xenotransplantation. Transpl Int 38:13867. doi: 10.3389/ti.2025.13867
Received: 29 September 2024; Accepted: 09 January 2025;
Published: 12 February 2025.
Copyright © 2025 Galdina, Puga Yung and Seebach. This is an open-access article distributed under the terms of the Creative Commons Attribution License (CC BY). The use, distribution or reproduction in other forums is permitted, provided the original author(s) and the copyright owner(s) are credited and that the original publication in this journal is cited, in accordance with accepted academic practice. No use, distribution or reproduction is permitted which does not comply with these terms.
*Correspondence: Jörg D. Seebach, Sm9lcmcuU2VlYmFjaEBodWcuY2g=
†ORCID: Viktoriia Galdina, orcid.org/0009-0008-7684-8688; Gisella L. Puga Yung, orcid.org/0000-0002-2283-7798; Jörg D. Seebach, orcid.org/0000-0001-5748-4577